"Clean Energy Transition" Part 4
Reassessing the global feasibility of nuclear power and mining the requisite critical minerals to transition all fossil fuels to renewables.
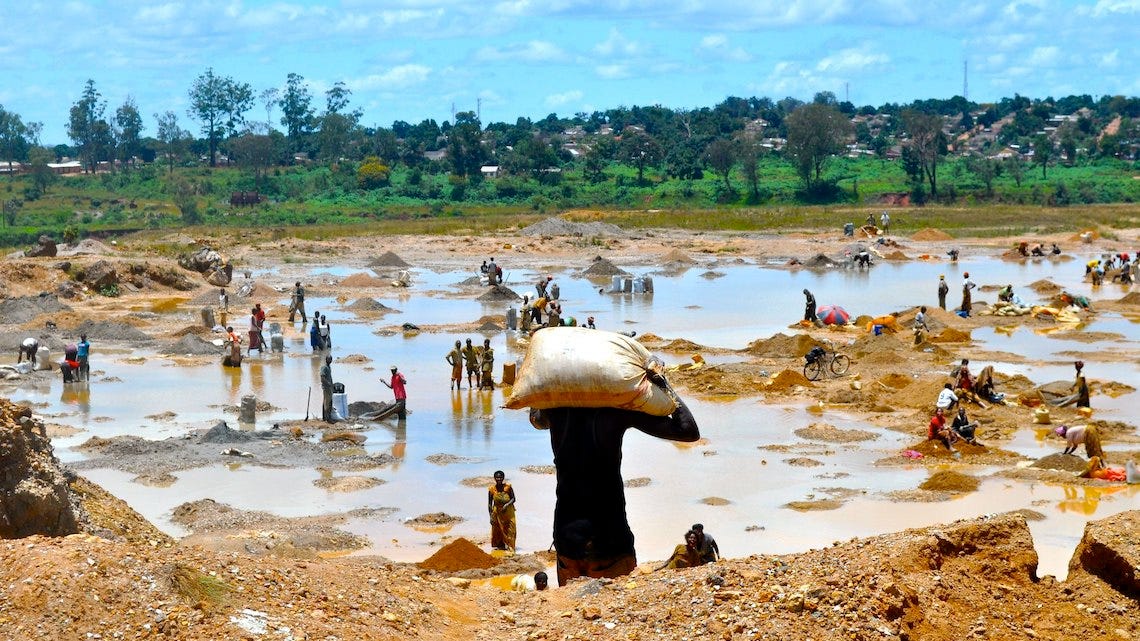
We are now at the pointy end of our discourse on the 2050 “Clean Energy Transition” conversation. In Part 1 we discussed the feasibility for Australia to go it alone and transition to 100% renewable energy sources. We saw that the task came with a AU$1.13 trillion price tag and the logistical impossibility to build enough renewable power plants by 2050 to supply Australia’s requisite 1,600 terawatt hours of annual energy needs currently supplied by fossil fuels.
In Part 2 we looked at the global picture: 47,000 additional terawatt hours of electrical energy would be required to transition every coal, oil and gas fuel application, be it power, industry or transportation, to renewable equivalents. That, too, was implausible by 2050. The details of that calculation are in the Scenario F section of this article.
In Part 3 we discussed three different energy alternatives to transition the global transportation sector away from oil in particular: EVs, hydrogen fuel cells and/or biofuels. Each system had its strengths and weaknesses. EVs were ideal for short range transportation; hydrogen for long-range; and biofuels for aviation. However, no matter what system or combination of systems we choose, the world must build more power plants to satisfy the increased power demand of EV and hydrogen economies. Biofuels were largely unviable. The idea of dedicating 40.31 million square kilometers of cropland and global forest to produce the necessary biofuels from soybean and corn for existing combustion motors was not viable in the slightest. It remains my firm suspicion that all three of these scenarios cannot be done and will not be done, especially not by 2050 as the globalist renewable-power investors want us to believe.
In this final installment of this series, we will return to Simon Michaux’s own hybrid proposal in his 2021 report, “Assessment of the Extra Capacity Required of Alternative Energy Electrical Power Systems to Completely Replace Fossil Fuels” (chapter 26, pp. 627-655). Here we must touch on two new issues. First is the inclusion of nuclear power in any reasonable or realistic discussion to meet Net Zero climate targets. At potentially 12.8 terawatt hours per plant per year, nuclear offers some of the biggest bang for buck in the carbon-zero base-load energy sector. Excluding nuclear from climate agenda goals for ideological reasons is nonsensical. Second is the acquisition of the raw materials necessary to construct the Net Zero, carbon-neutral, renewable energy world of the future. In this regard I will draw on the assessment of one other important document: the IEA’s 2022 revised report, “The Role of Critical Minerals in Clean Energy Transitions.” Together with Michaux, these two reports should settle the question of whether 2050 Net Zero transition targets are truly feasible or not.
Scenario E: The Nuclear Question
[NOTE: See my pinned comment below for an interesting critique of Michaux’s poorly argued Nuclear Question. I will otherwise leave this section as I originally wrote it.]
Part 2 and Part 3 made it abundantly clear that the world is going to need a lot more electrical energy production to make any clean energy transition plausible. Estimations ranged from an additional 10,000 terawatt hours of annual electrical power production required for a 100% EV transportation fleet; 17,000 terawatt hours to replace all coal, gas and oil power generation; 30,000 terawatt hours for a functional 100% hydrogen economy; or an impossible 194,000 terawatt hours for a 100% algal biodiesel future. All this new power must come from somewhere, that is, new power plants to be constructed. But those new plants cannot be energy-dense fossil fuels like coal, gas or oil. In most scenarios, solar and wind alone were insufficient to meet the new demands. Neither were there enough plausible sites for new hydroelectric dams, the only ‘energy-dense’ renewable power source.
As it now stands, nuclear power remains beside hydroelectric as the sole monolith for ‘clean’, carbon-neutral energy production (notwithstanding the issue of nuclear waste). So how viable is the nuclear question? Would humanity be able to reasonably transition our future “clean energy” requirements to 100% nuclear power?
Michaux dealt with this topic at significant length in Scenario E (chapters 24-25, pp. 499-626) of his report. Calculating estimations for increases in the global nuclear fleet were not easy and entailed a significant number of complex assumptions such as: expansion of uranium mining and nuclear fuel processing; nuclear plant construction speeds; decommissioning rates of old plants/technology; and storage options for spent nuclear fuels of various risk levels. The entire nuclear cycle is represented as follows:
Briefly summarised, the important factors of the nuclear equation included:
The global number of nuclear power plants and their annual electricity production. This was calculated at 443 plants (2019 data), producing approximately 2,657.2 terawatt hours annually (Michaux, 2021, pp. 504-506).
Generation II nuclear plants (90% of current nuclear fleet) must be phased out and replaced with more efficient Generation III+ plants which require 29.9 tonnes of processed uranium fuel per year per plant to generate 11.2 terawatt hours of annual electricity each (Michaux, 2021, p. 566).
Global demand for nuclear-fuel grade uranium was calculated at approximately 59,200 tonnes per year (2019 data; Michaux, 2021, p. 507).
Future global demand for nuclear-fuel grade uranium was calculated at between 56,640 to 100,224 tonnes per year (by year 2040; Michaux, 2021, p. 508).
Global production of fuel-grade uranium was 54,224 tonnes per year (2019 data); historical total mined uranium production has been 2,965,314 tonnes, approximately 40,000 tonnes per year (1945 through 2019; Michaux, 2021, pp. 511-512). The majority of the world’s supply of uranium comes from Kazakhstan, Canada and Australia, with Brazil, Namibia, Mongolia and Morocco representing significant future resource locations.
Future global production of fuel grade uranium was estimated between 43,000 and 73,000 tonnes per year (by year 2040; Michaux, 2021, p. 513).
Remaining global uranium reserves (all categories) were estimated at a grand total 23,717,600 tonnes (Michaux, 2021, p. 525). This calculation included speculative uranium recovery from other mining operations, e.g. phosphate, copper, and gold, as well as the reprocessing of uranium tailings and spent fuel rods.
Approximately 11,000-18,000 tonnes of spent nuclear fuel waste is produced per year (Michaux, 2021, p. 538); a total of 370,000 tonnes have been produced since the commencement of civilian nuclear power in 1945, of which 124,000 tonnes has been reprocessed for reuse; 246,000 tonnes remain in storage with no permanent long-term disposal solution, of which 172,200 tonnes (70%) remains in power-cooled storage to dissipate radioactive heat.
Michaux’s first nuclear thought experiment (pp. 566-578) was if the world commenced construction of two (2) new Generation III+ nuclear power plants per decade, starting in 2025, while also simultaneously phasing out Generation II reactors for Gen. III+ reactors. In this slow scenario, comparable to current nuclear power plant expansion rates, the global nuclear fleet would reach a total output of 4,477 terawatt hours annual capacity by 2325. All of the world’s 23.7 million tonnes of uranium resources would be depleted by that year in this model, at which time there would be 503 Generation III+ power plants to decommission and 4.2 million tonnes of spent nuclear fuel (all categories of radioactive risk, from very low to high) in powered cooling without any more nuclear reactors to power that cooling for another decade.
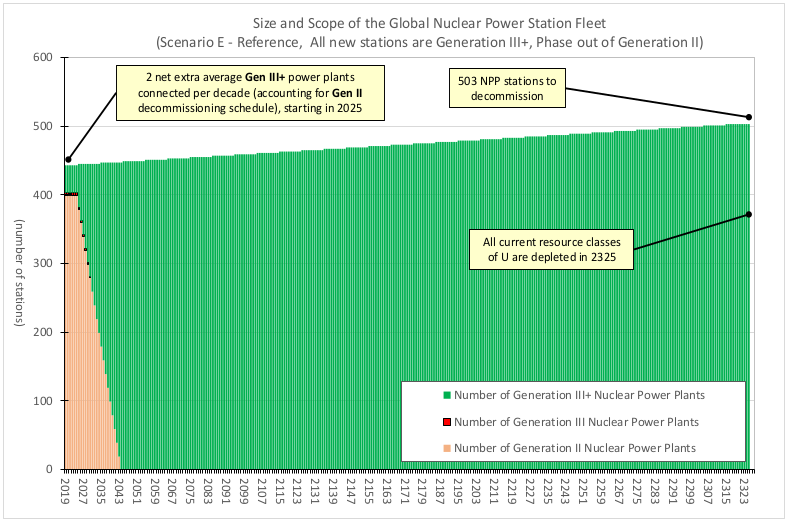
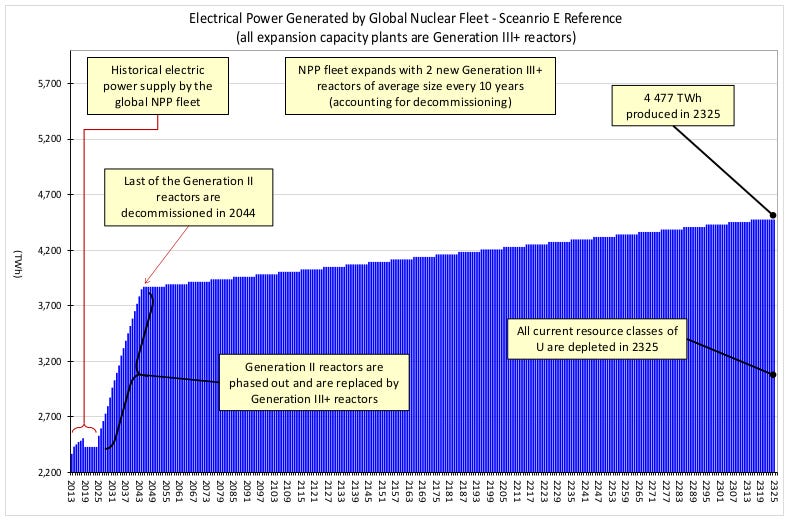
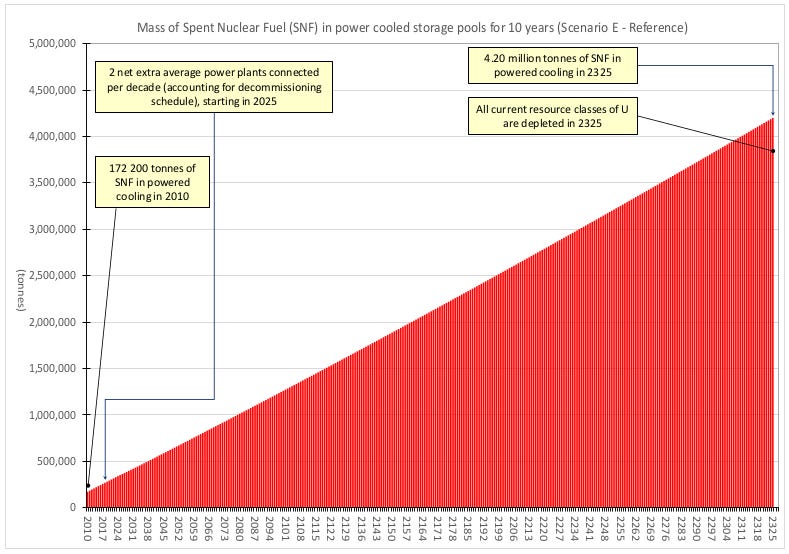
Overall, this reference Scenario E would result in a modest doubling of world nuclear power generation by year 2325, at which point the world’s uranium would be exhausted. The issue of dealing with 4.2 million tonnes of spent nuclear fuel in potentially unpowerable cooling ponds (because all the world’s nuclear would be decommissioned at that time) is a significant future issue that must be factored into this scenario. Better means of nuclear waste separation and disposal are necessary (i.e., deep earth storage).
In a second thought experiment (pp. 579-592), Michaux assumed that the world commenced an “energy industrial emergency” in 2021 and enhanced the construction rate of these 11.2 terawatt hour Generation III+ nuclear reactors from two per decade to 250 per decade (25 per year). New reactors begin power operation a mere 5 years after the commencement of their construction. Twenty Gen. II reactors would be decommissioned and replaced with Gen. III+ per year from 2021 through 2047, when all Gen IIs would have been upgraded to Gen. III+. In this industrially hastened scenario the world’s supply of uranium, 23.7 million tonnes, would be exhausted by year 2101, having been consumed in less than 80 years by the 2,345 Gen. III+ nuclear reactors constructed during that time. Just prior to nuclear fuel shortages in 2101, those reactors would have been providing 25,663 terawatt hours of electricity per year to the global grid.
Michaux allowed for the possibility of permanent nuclear waste disposal in deep underground storage facilities in this hastened scenario, making his spent nuclear fuel outcomes vastly different to the previous iteration. By 2101 there would be:
444,609 tonnes of hot nuclear waste requiring power-cooled storage for another 10 years;
1.18 million tonnes of very low level waste (VLLW) in landfill;
2.81 million tonnes of low level waste (LLW) in near-surface (30m depth) storage for 300 years;
66,218 tonnes of intermediate level waste (ILW) in deep underground (90-300m depth) repositories for 10,000 years;
2,437 tonnes of high level waste (HLW) in very deep underground (500-1000m depth) repositories for 100,000+ years.
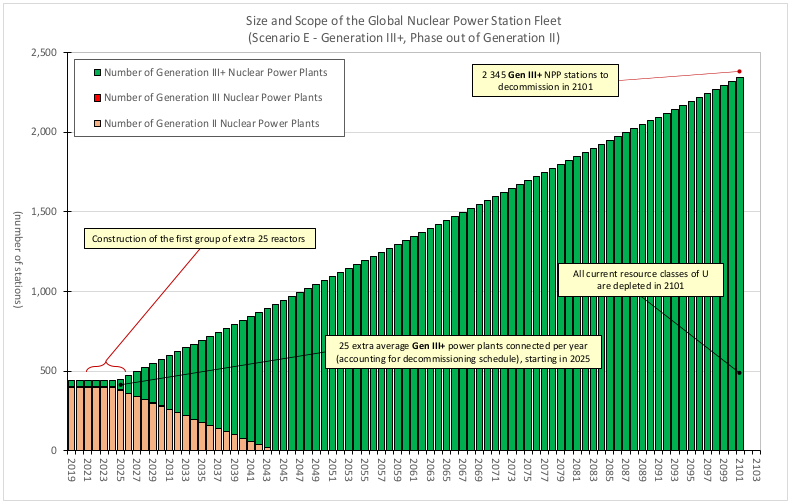
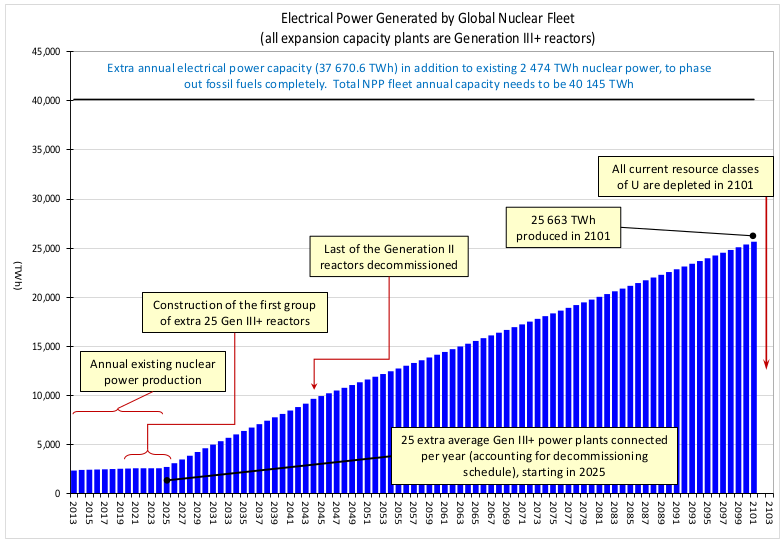
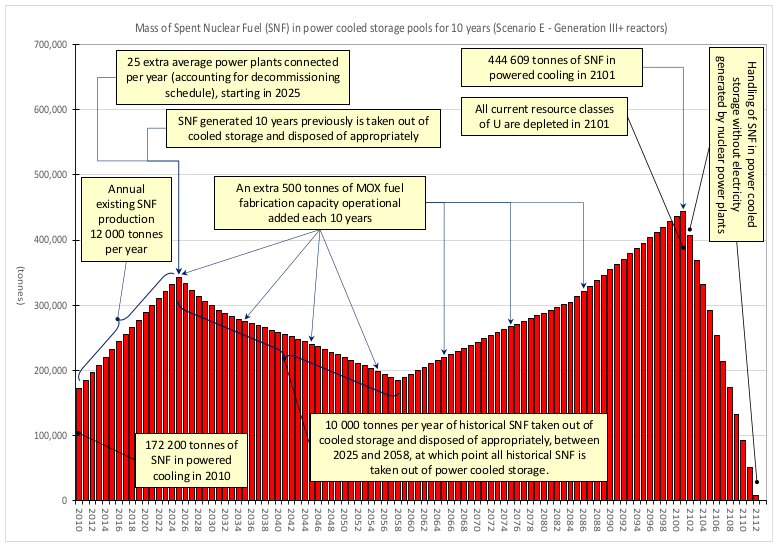
Two more nuclear thought experiments were conducted in order to be thorough. One used Gen. II reactors to accomplish the same task as the previous industrially-hastened scenario (Michaux, 2021, pp. 593-605), a suggestion of at least one European member of parliament. It resulted in the world’s uranium running out by 2095 at the hands of 2,195 Gen. II reactors and the nuclear fleet generating 16,422 terawatt hours of electrical power at its zenith just prior to the collapse of uranium supplies.
The other scenario used conceptual Gen. IV Transverse Wave Reactors (Michaux, 2021, pp. 606-622), which may reuse spent nuclear fuel as their fuel. Michaux assumed that 10 such reactors were added to the global power grid every year from 2030, and they could reuse up to 75% of their spent fuel as new fuel. In this Gen. IV scenario, the world’s uranium would be exhausted by 2194 at the hands of 4,375 Gen. IV plants which would have been operating at 20,977 terawatt hours by year 2100, and 48,100 terawatt hours of electrical capacity prior to uranium fuel shortages commencing in 2194.
To summarise Michaux’s findings, we have four nuclear scenarios that were explored:
503 Gen. III+ reactors constructed at approximately current global rates, producing up to 4,477 TWh annually and exhausting uranium by 2325;
2,345 Gen. III+ reactors constructed at ‘energy emergency’ rates, producing up to 25,663 TWh annually and exhausting uranium by 2101;
2,195 Gen. II reactors constructed at ‘energy emergency’ rates, producing up to 16,422 TWh annually and exhausting uranium by 2095;
4,375 conceptual Gen. IV reactors constructed at ‘energy emergency’ rates, producing up to 48,100 TWh annually and exhausting uranium by 2194.
Only the Gen IV reactor scenario shows any plausibility in alleviating humanity’s future energy crisis. However, that scenario is entirely based on turning a conceptual technology into reliable reality; this has not yet happened, Gen. IV Transverse Wave reactors remain hypothetical only. The other options either produce too little overall energy to cover the global EV/hydrogen transition, are logistically implausible (Michaux’s ‘nuclear energy emergency’ is a civil engineering nightmare), or exhaust uranium too quickly (by 2100, give or take 5 years). Overall, a 100% nuclear-powered global electricity grid is not viable. Other sources of electricity are needed to assist nuclear to bear the brunt of humanity’s expanding energy needs if indeed a ‘clean transition’ to EV and hydrogen transportation fleets is in humanity’s best interests. With that in mind, let us turn to Michaux’s own future energy scenario.
Scenario F: Michaux’s Hybrid Proposal
As we saw in Part 3, a clean transformation of the transportation sector to each alternative (EVs, hydrogen fuel cells or biofuels) came with unique advantages and disadvantages. EVs excelled for short-range applications and were thus ideal for urban operation of short range vehicles like motorbikes, cars, light delivery vans, buses, and short range ferries. Hydrogen fuel cells were preferred for long range applications, especially freight transport: long-haul trucks, rail and shipping. Biofuel was only suitable to replace jet fuel, but even that seemed implausible due to the necessary land area and irrigation quantities involved. Aviation was thus unable to reliably transition to any suitable non-fossil fuel alternative. Michaux calculated his global, hybrid-model transportation fleet transition as follows:
1.39 billion EVs for short-range applications requiring 6,158.4 terawatt hours of new electrical power annually and 282.6 million tonnes of batteries (Table 26.4).
29.13 million hydrogen fuel cell freight vehicles (trucks, trains and ships) requiring 11,553.6 terawatt hours of electrical power annually for the electrolysis of 200.1 million tonnes of hydrogen (Table 26.6).
The global aviation fleet is gutted, retaining “a small proportion only” (Michaux, p. 634) able to be sustained by small-scale biofuel production.1
TOTAL additional energy required: 17,712 terawatt hours (Table 26.7).
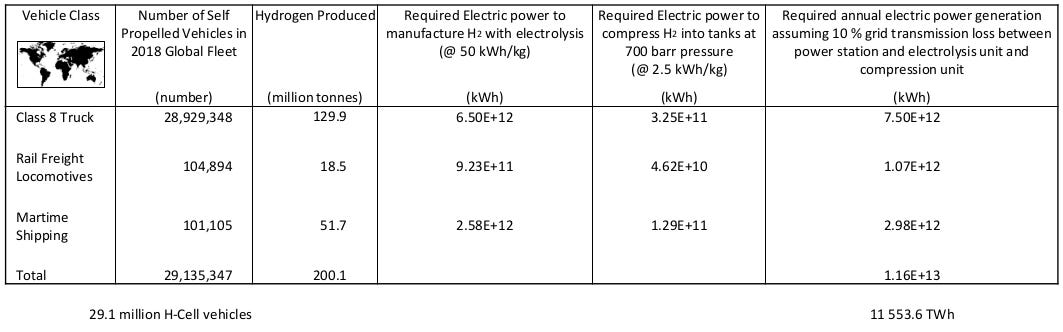
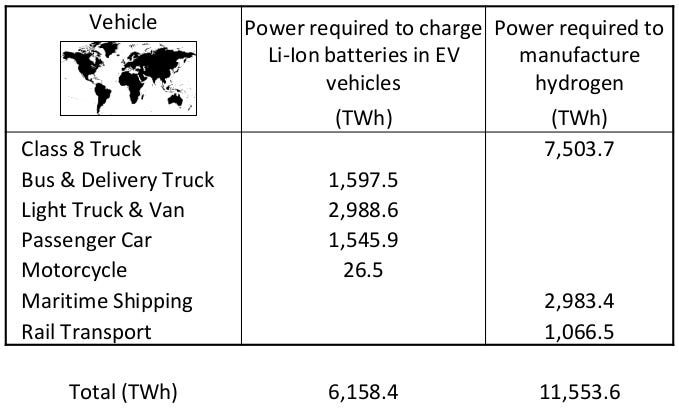
In terms of Michaux’s overall power grid transition, he highlighted the important role that nuclear and hydro play in any viable ‘clean’ transition. While neither nuclear or hydro alone could possibly wholly replace coal, oil and gas in a clean energy future, both systems must be considered for their reliable base-load power which is a necessity for many modern industrial, commercial and residential applications. Nuclear power should continue at current development rates, rather than be scaled up, so its mined uranium and thorium fuel sources can last 300+ years rather than 80 years.
The inherently intermittent power of solar and wind must be buffered against by the use of large battery banks able to store and release peak power on demand. Biofuels may replace some, but not all, petroleum for plastic production; biomass feedstock can be used to replace some specialised high temperature coal operations. Lastly, gas use for chemical fertiliser production has no viable alternative; industrial agriculture must be phased out entirely and replaced with small-scale regenerative farming practices, especially those that reclaim land degraded by more than a century of industrial-chemical agriculture. Overall, Michaux’s hybrid solution for a clean energy transition of the global power grid totaled 19,958.6 terawatt hours of energy to be sourced from non-fossil fuel power plants:
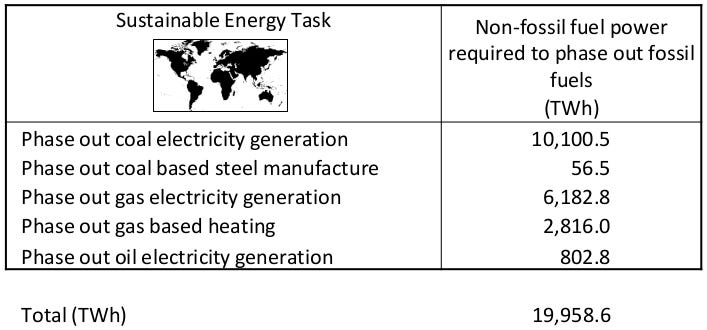
Together, Michaux’s hybrid scenario’s estimation of our future clean energy transition needs is clear and unambiguous:
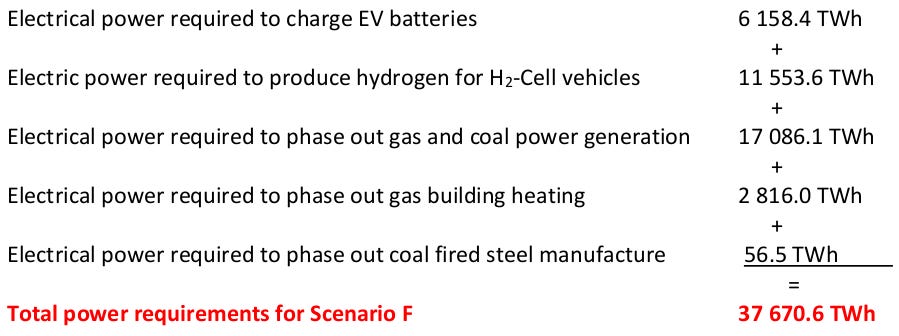
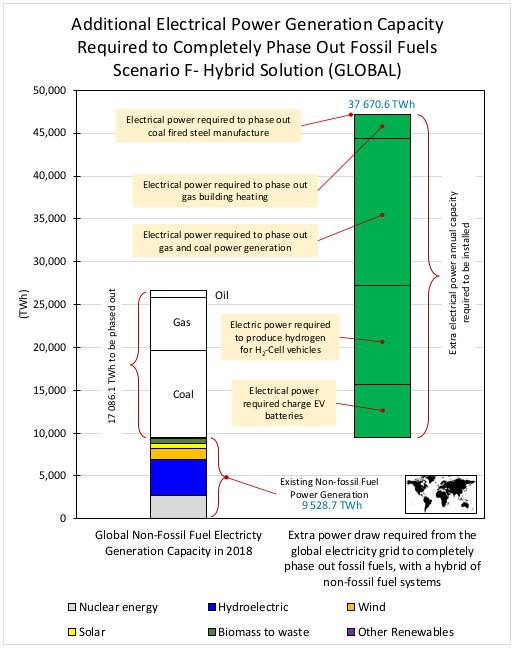
Of course, Michaux calculated the number of new, non-fossil fuel power plants that would be required to supply that 37,670.6 terawatt hours of electricity to keep the world moving by 2050: 221,594 new non-fossil fuel power plants! If you are wondering why this number keeps changing in each article, it is because different scenarios put forward by Michaux are utilising different parameters and assumptions. Michaux’s breakdown of these Scenario F power plants, following 2018 ratios, was as follows:
834 new nuclear plants;
12,504 new hydroelectric dams;
63,445 new wind farms;
69,291 new solar farms;
282 new solar thermal farms;
609 new geothermal plants;
74,628 new biowaste/biomass plants.
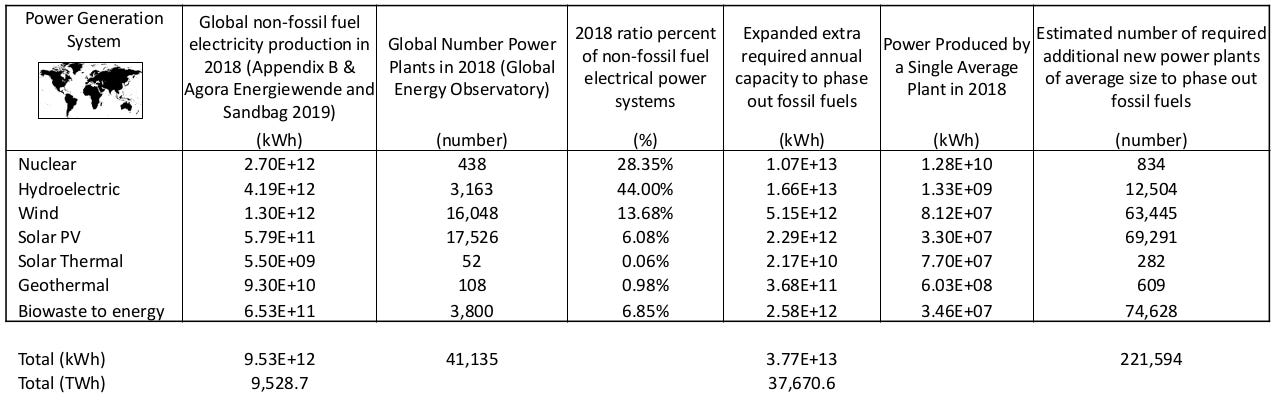
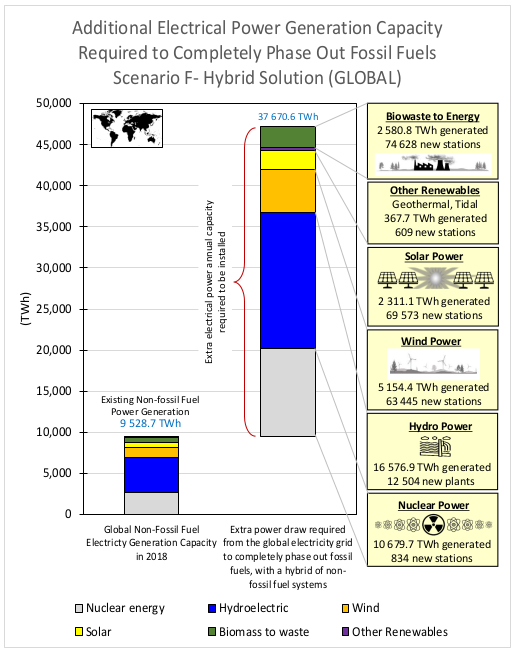
The overall transition footprint for Scenario F was encapsulated in this one graphic:
Of course, not calculated by Michaux was the rate of deployment if these 221,594 power plants were to be delivered by our Net Zero 2050 deadline (starting 1 Jan. 2022). In honour of Kenneth Schultz in Part 1 we have:
2.5 nuclear power plants per month;
37 new hydroelectric dams per month;
189 new wind farms per month;
206 new solar farms per month;
1 new solar thermal farm per month;
2 new geothermal plants per month;
222 new biowaste/biomass plants per month.
If building all of that within the next 28 years was not enough to make your head spin, things are about to get worse.
NOT ENOUGH MINERALS
Now we come to the crux of this article (and I apologise for the necessary detour to set the Scenario E and F benchmarks). How much critical minerals are required to effect that global power, heating, industrial and transportation transition to supply that extra 37,670.6 terawatt hours of global annual power Michaux hypothesised in his Scenario F? Is it actually possible to build 1.39 billion EVs, supply hydrogen for 29 million hydrogen trucks, 104,000 hydrogen trains, 101,105 hydrogen ships, and the additional estimated 221,594 power plants (and necessary surge/peak mitigation power storage) to power them all?
Uranium for Nuclear
We already discussed the uranium question and found that it was not possible to transition the world to 100% nuclear power without exhausting literally every possible source of uranium by year 2095 with Gen. II reactors, by year 2101 with Gen. III+ reactors, or by year 2194 with unproven and conceptual Gen. IV reactors. Keeping nuclear fleet expansion at its current rate would see 503 reactors well-fueled up to year 2325, providing approximately 4,400 terawatt hours of annual electricity generation. Furthermore, the nuclear problem included having to deal with powering the cooling ponds of several hundred thousand tonnes of dangerous nuclear waste for another 10 years at the time global uranium reserves run out without having any remaining nuclear power to do so. Thus, uranium’s destiny in the critical mineral picture is for it, too, to go the way of fossil fuels and disappear forever.
EV Batteries
Michaux’s helpful benchmarks give us ample opportunity to guess at the quantities of refined minerals required to effect an EV transition on the scale he envisaged in Scenario F. One only needs the average mineral quanities/ratios in a lithium-ion battery:
Leaving aside Michaux’s unexpected omission of manganese (Mn, the ‘M’ in NMC 811; I believe he may have mistakenly utilised the chemistry for NCA —Nickel, Cobalt, Aluminium— batteries in figure 26.8 above), we have the general mineral proportions for the average 230 Wh/kg lithium-ion battery. Thus, it can be expanded out to the estimated fleet of 1.39 billion EV vehicles requiring that estimated 282.6 million tonnes of batteries calculated above in Table 26.4:
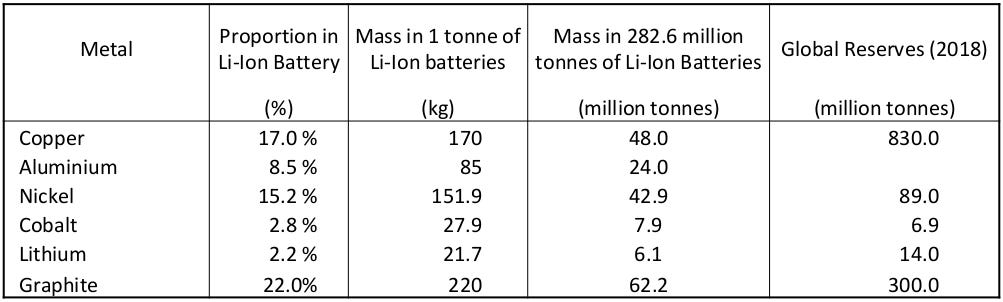
Surprisingly, the test passes at every mark except cobalt, which was 1 million tonnes short. There are indeed enough minerals to effect a global transition of the short-range vehicle fleet to EVs!
However, one of two serious complicating factors in this picture is the annual production of these precious metals. This is where a hopeful clean transition to EVs takes a drastic turn for the worse:
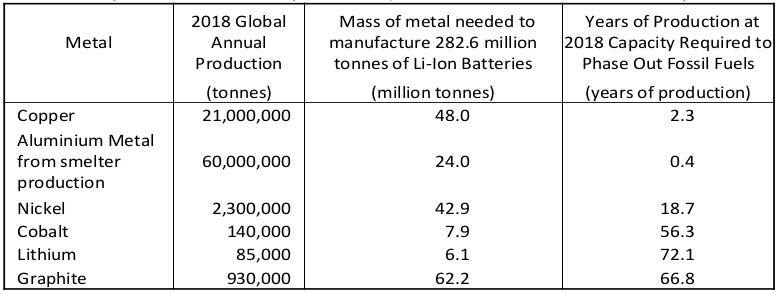
That’s right. If we divert 100% of the world’s copper production to EV batteries, we can stockpile enough copper to produce 282.6 million tonnes of batteries within 2.3 years. If 100% of the aluminium was likewise diverted, it would take 5 months to amass the required stockpile for lithium-ion battery cathodes. But nickel production would take 18.7 years at a 100% diversion rate, cobalt production 56.3 years at the same, lithium 72.1 years and graphite 66.8 years. Mining production of these latter four minerals needs to increase markedly, especially if 2030 or 2050 deadlines are to be met. That means more mining, more refining, more smelting and more processing of these materials at a time when green politics is whining and crying out for less. But it gets worse.
The second complicating factor is that EV battery plausibility lasts for only a single generation of batteries. After 8-10 years of use, that crop of 282.6 million tonnes of batteries can no longer cycle or charge. Thus, the entire process must be repeated for another 282.6 million tonnes of new batteries. This is such a crucial point that I will quote Michaux (p. 650) at length for emphasis:
Each of these lithium ion batteries will have a useful working life of 8 to 10 years only (IEA 2019b). So, 8-10 years after manufacture, new replacement batteries will be required, from either a mined mineral source, or a recycled metal source. As less than 0.5% of the current transport fleet is EV, these batteries cannot be resourced from recycling. The first generation has yet to be manufactured. Once that first generation is worn out, they can be recycled, which has its own challenges. [This calculation] shows only what is required to make one battery for each vehicle. It does not allow for mass estimations for other batteries like mobile phones, computers, or appliances. Nor does it allow for other demand applications for these metals outside battery manufacture, like metal alloy production, or ammunition production.
In theory, there are enough global reserves of nickel and lithium if they were exclusively used just to produce li-Ion batteries for vehicles. To make just one battery for each vehicle in the global transport fleet (excluding Class 8 HCV trucks), it would require 48.2% of 2018 global nickel reserves, and 43.8% of global lithium reserves (Source: USGS Mineral Statistics). There is not enough cobalt in current reserves to meet this demand and more will have to be discovered in exploration.
In practice, this will not work due to other demand application requirements, and that this represents only one generation of batteries of the current vehicle fleet. Every ten years from that point onwards, the same mass requirement would be needed all over again to produce the next generation of EV battery.
Suddenly, the EV dream disappears in a wisp of lithium smoke. Every 8-10 years the world’s 1.39 billion-strong EV fleet calculated in Scenario F will require 282.6 million tonnes of batteries. It cannot be done and it will not be done. Michaux drove this point home (pp. 653-655) when he eviscerated various WEF and IEA reports glowingly promoting how 30% of the world’s vehicle fleet would be EV by 2030, or how a certain percentage of the global electrical grid would be supplied by CO2-free options by those dates. Those global powerbrokers could not even get their numbers right because they did not properly calculate the true magnitude of the task at hand!
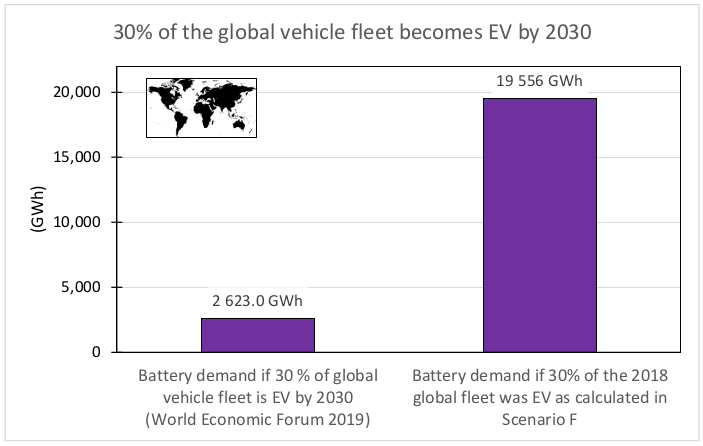
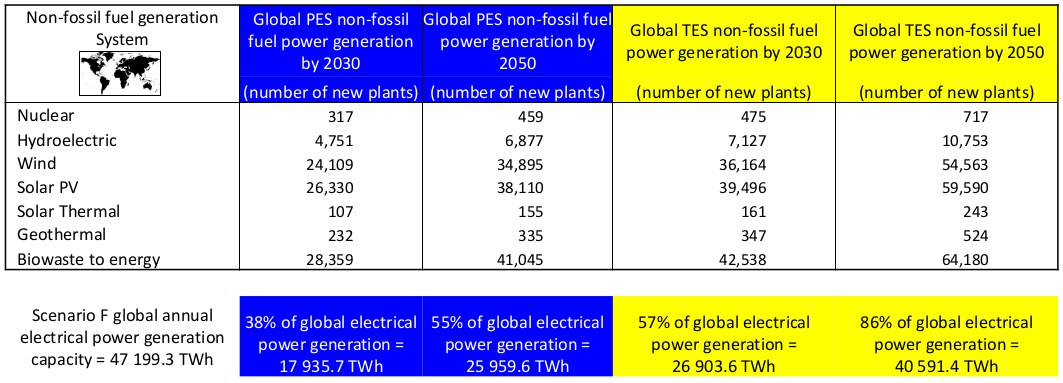
Michaux was very benign when he concluded (p. 655): “This suggests that the 2050 climate neutral target (European Commission 2019) task is much greater than current planners understand.” 282.6 million tonnes of batteries every 8-10 years from now until our eternal clean energy future! It cannot be done and it will not be done!
Lithium-ion Power Mitigation Storage
One underappreciated component of the renewable debate is the necessity for power storage to accompany solar and wind. Those renewables are inherently intermittent; their power output varies by the minute and by the season. Therefore, when a global electrical grid transitions to intermittent clean renewables like solar and wind, a necessary storage buffer is required to supply surging demand at short notice.
Michaux pointed out that, as of 2018 data, 98% of the world’s electricity storage is kept in hydroelectric pumped potential. That is to say that when power grids are hit with a surge in demand (such as daily peak between 2pm-8pm), hydroelectric dams dump water through their turbines to supply that surge and pump it back during an off-peak time for reuse at the next peak period. Other current peak surge power supply options include gas and oil power plants which can easily be turned on and off as demand requires. Hydroelectric may not be able to supply this future power storage requirement alone due to dam site limitations, thus battery storage like the 100 MW South Australian Hornsdale Power Reserve become necessary to mitigate this aspect of the future clean transition global power grid. Michaux utilised this Australian 100 MW battery storage station as the basic design for his assumptions to calculate the necessary number of such stations required to supply 1 month’s worth of on-demand electrical energy for the transitioned future grid, which he calculated at 574.3 terawatt hours of mitigation capacity:
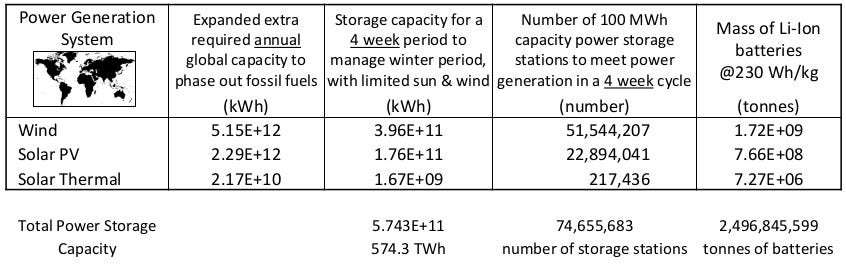
Suddenly the EV battery proposition jumped from a plausible 282.6 million tonnes of metals for one generation of EV batteries to 74.6 million 100 MW battery banks requiring an additional 2.5 billion tonnes of batteries, for a grand total of 2.77 billion tonnes of batteries, plus 2.77 billion tonnes more every 8-10 years thereafter to replace the old ones. Do you think there are enough critical battery minerals for that?
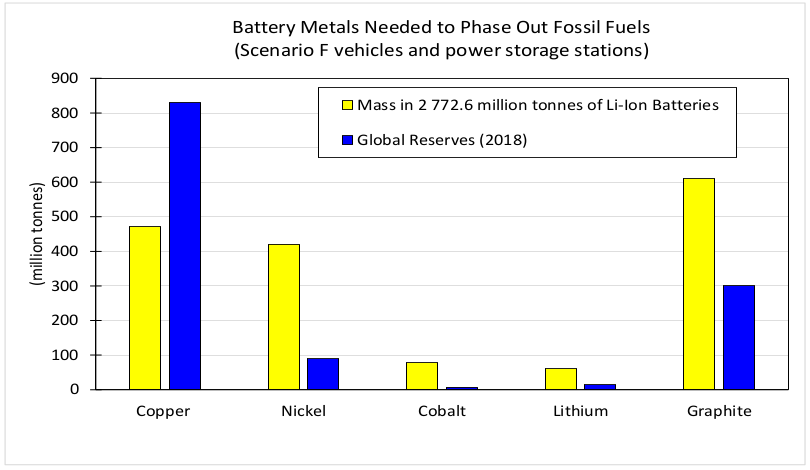
Only copper made par for the first generation only. If we rape the entire planet of literally every last atom of these transition-critical metals, and then proceed to outer space to rape every asteroid, moon, planet, Uranus and the sun for everything they’ve got too, maybe we will have enough for the ‘clean transition’ dream. It’s Unobtainium (Avatar reference) on steroids. It cannot be done and it will not be done!
Vanadium Redox Flow Battery (VRFB) Mitigation Storage
Michaux never included vanadium redox flow batteries (VRFBs) for static energy storage in any of his calculations. These are an allegedly promising option for battery storage power due to their expected 25-30 life spans, 200,000+ potential recharge cycles and 100% electrolyte recyclability. So in order to explore their potential to replace 2.5 billion tonnes of lithium-ion battery storage farms I went and did the hard work myself. Examples of modular vanadium battery systems such as Cellcube and VSun (the latter did not respond to any of my questions) abound; other large VRFBs are also possible (see Minke 2017 below).
Each VRFB consists of individual battery cells through which a vandium-sulfuric acid electrolyte is pumped alongside a bipolar plate and ion-permeable membrane, resulting in electrical charge separation at the electrodes which can be collected and put to use like any other electric battery. However, their energy density is low, in the range of 20-30 watt-hours/kg (lithium ion exceeds 200 Wh/kg; diesel fuel was 12,750 Wh/kg). An entire collection of multiple cells joined together is called a stack, and multiple stacks can be combined to provide a particular power output for a certain time, e.g. 10 MW for 10 hours (100 MWh).
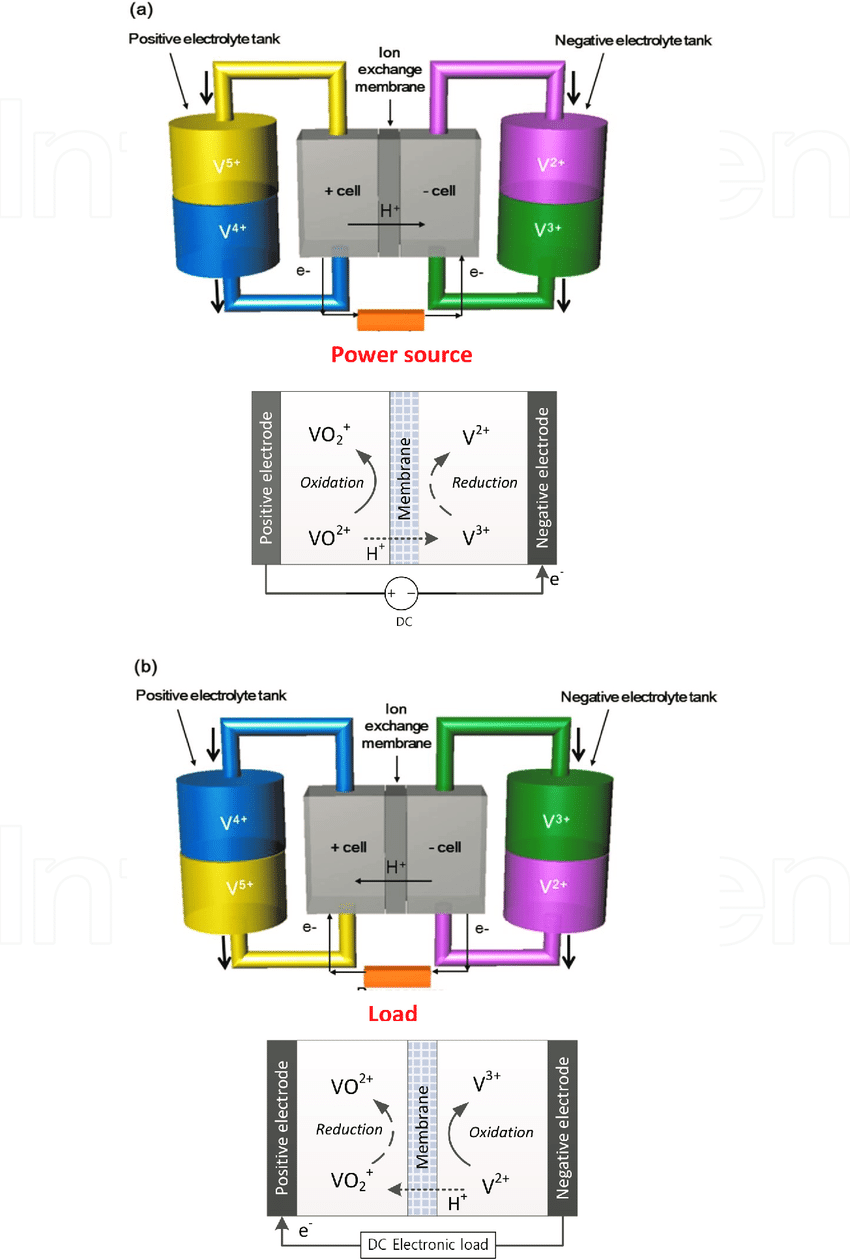
According to one particular journal article, VRFBs utilise approximately 1.6 mol of vanadium per 1000 ml (1 litre) of solute (sulfuric acid), although each vanadium battery has electrolyte concentrations optimised for its own ambient site conditions.2 Another paper estimated the overall cost of a 10 kW/ 120 kWh vanadium battery stack (8,257 litres) at approximately €130,000, of which the vanadium in the electrolyte accounted for €19,816; the entire electrolyte solution accounted for 31% of the overall system cost.3
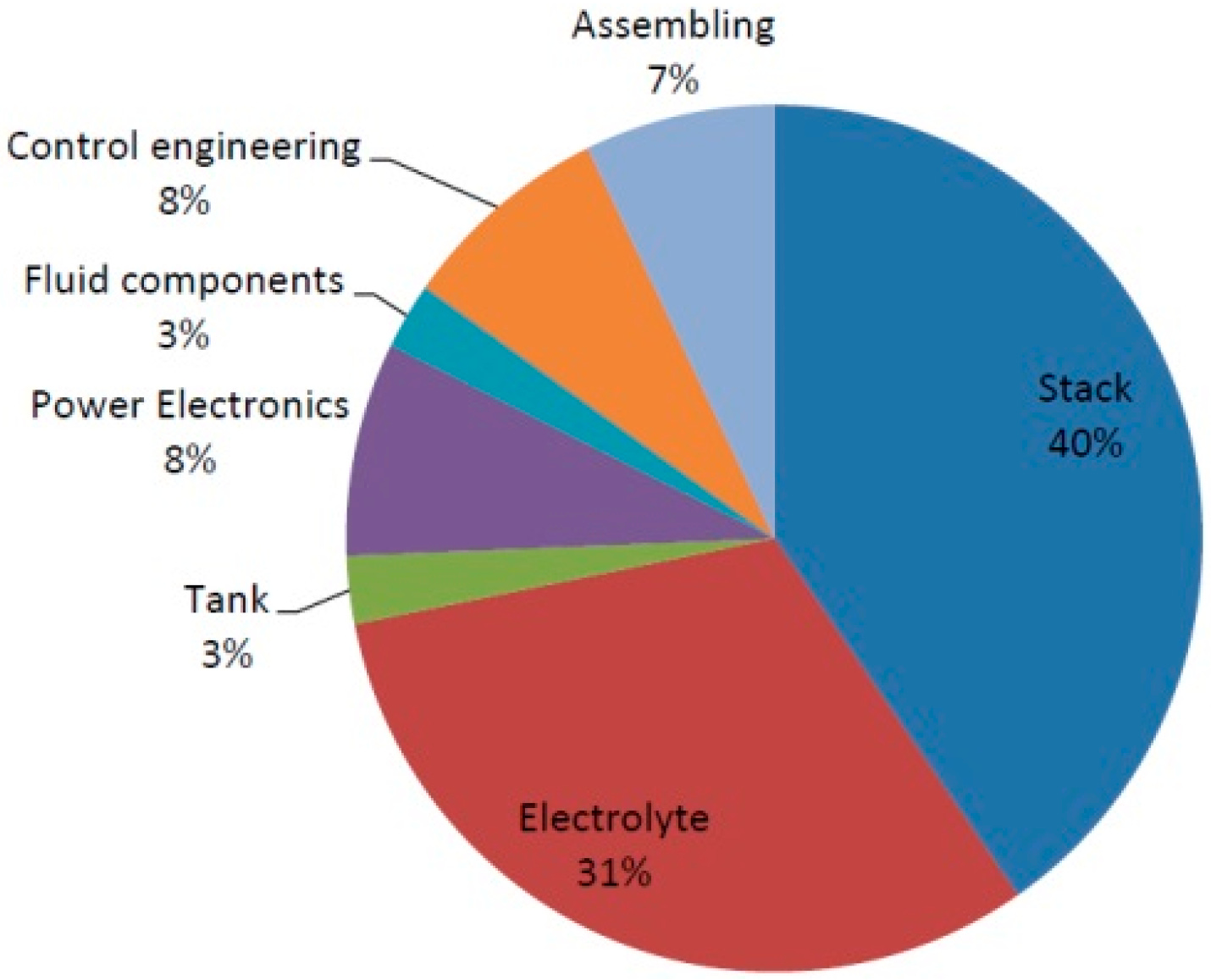
A third paper estimated the costs of large-surface area VRFB technology suitable to supply megawatt hours of energy, which is the kind of application suited to grid power mitigation under consideration here.4 They calculated 82 grams of vanadium per litre of electrolyte (or 82 kg per cubic metre) which costs approximately €1630.10 per cubic metre of battery volume. The other components of VRFB electrolyte solutions, sulphuric acid and water, were €19.60 and €1.5 per cubic metre respectively:

Given the suitability of this large VRFB system for grid mitigation needs, this is the VRFB type that I chose to run with for power output, cost and mineral calculations. Minke et al. (2017b) supplied these helpful charts:
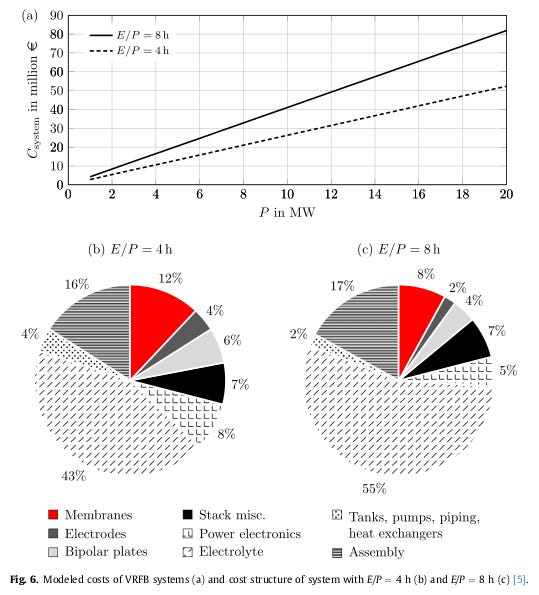
At the top we have a graph charting cost versus power output of a large VRFB system. A 10 MW VRFB stack able to supply 4 hours of electricity would cost approximately €26 million (dotted line). A 10 MW VRFB stack able to supply 8 hours of electricity would cost approximately €40 million (solid line). A 10 MW system which operates for up to 8 hours would be 80 MWh. A 10 MW system which operates for 4 hours would be a 40 MWh system. It would be possible to have a 10 MW system operating for up to 10 hours, or a 100 MWh system, or another combination such as a 12.5 MW system discharging for 8 hours, also 100 MWh.
For ease of comparison with Michaux’s lithium-ion battery banks, we will run with this latter system: a 12.5 MW VRFB stack discharging for up to 8 hours to supply up to 100 MWh. Minke et al. (2017, p. 110) noted that a 4 MW VRFB system would require a 190 cubic metre (approx. 190,000 litre) electrolyte tank, or roughly 47.5 cubic metres per MW. Our system is 12.5 MW, so we require 594 cubic meters of electrolyte per 12.5 MW stack. Each cubic metre of electrolyte would contain 82 kg (0.082 tonnes) of vanadium, 392 kg (0.392 tonnes) of sulfuric acid and 876 kg (0.876 tonnes) of water. Thus, each 12.5 MW stack needs 48.7 tonnes of vanadium, 232.85 tonnes of sulfuric acid, and 520 tonnes of water to operate. Further, a 250 kW (0.25 MW) stack consists of 75 individual battery cells (Minke et al., 2017b, p. 108); thus, our 12.5 MW stack is assumed to consist of 3,750 cells each. Each cell requires one ion exchange membrane made from either Nafion™5 or SPEEK6 (Sulfonated poly ether ether ketone); two graphite felt electrodes;7 and one 2.7 square metre carbon-polymer (polypropylene) bipolar plate.8 A 250 kW stack of 75 cells also requires two 250 kg copper current collectors; thus for each 12.5 MW stack we assume two 12.5 tonne copper current collectors. Let us assume the other costs parallel the E/P = 8h pie chart in Figure 6 above.
Our overall VRBS mitigation storage to supply 574.3 terawatt hours of electrical power for up to 8 hours (down from Michaux’s 30 days) to accommodate intermittent solar and wind supply are:
5,743,000 (12.5 MW/100 MWh) VRFB stacks consisting of 21,536,250,000 cells (3,750 cells per stack);
3,411,342,000 (3.4 billion) cubic metres of electrolyte requiring:
2.797 million tonnes of vanadium (at 0.082 tonnes/cubic metre);
1,337.3 million tonnes of sulfuric acid (at 0.392 tonnes/cubic metre);
29,881.5 million tonnes of water (at 0.876 tonnes/cubic metre);
143.6 million tonnes of copper for the current collectors;
21.5 billion Nafion or SPEEK membranes to be manufactured;
21.5 billion carbon-polymer bipolar plates to be manufactured;
43 billion graphite felt electrodes to be manufactured.
The total cost, at approximately €50 million per 12.5 MW unit (Minke, 2016, Figure 6 above), would be €28,715,000,000,000 (28.7 trillion Euros).
A 2022 USGS Vanadium Mineral Commodity Survey cited 110,000 tonnes of global vanadium production. It would require 25.5 years of that production level to acquire the necessary vanadium for 5.743 million 12.5 MW/100 MWh vanadium battery stacks. That same USGS survey estimated 63 million tonnes of vanadium in the world. There is enough vanadium to do it, apparently. However, there is not enough manufacturing capability to make the other critical components of that many vanadium battery cells: 21.5 billion membranes, 21.5 billion bipolar plates (made from oil-based plastic!); and 43 billion graphite electrodes.
Vanadium redox flow batteries are thus found to be an unviable solution to supply a clean-transitioned EV, hydrogen and renewable world’s energy mitigation capacity. It cannot be done and will not be done. Even here a hybrid energy storage system to mitigate solar and wind intermittency is found to be necessary. Vanadium and lithium-ion battery parks will not be enough on their own.
Power Plants for All
Michaux excluded mineral calculations for his predicted 221,594 non-fossil fuel power plants by 2050. Fortunately, we can fill in the gaps from the IEA’s revised 2022 report, “The Role of Critical Minerals in Clean Energy Transitions.” Included in that report are the types and quantities of critical minerals required for certain power plants:
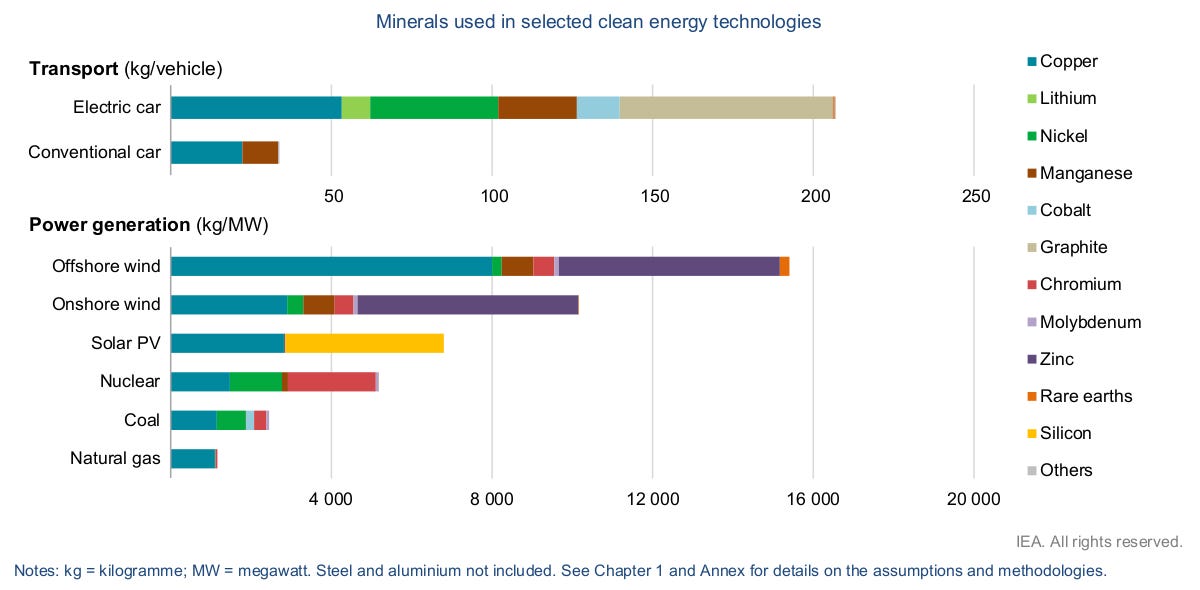
The above graph is somewhat difficult to read, so I will break it down into approximate quantities of respective critical minerals required in the construction of each power plant type (concrete, fibreglass and aluminium excluded):
Offshore wind:
Copper: 8,000 kg (8 tonnes/MW)
Nickel: 300 kg (0.3 tonnes/MW)
Manganese: 700 kg (0.7 tonnes/MW)
Chromium: 500 kg (0.5 tonnes/MW)
Molybdenum: 100 kg (0.1 tonnes/MW)
Zinc: 5,500 kg (5.5 tonnes/MW)
Rare earths: 300 kg (0.3 tonnes/MW)
TOTAL: 15,400 kg (15.4 tonnes/MW)
Onshore wind:
Copper: 2,900 kg (2.9 tonnes/MW)
Nickel: 400 kg (0.4 tonnes/MW)
Manganese: 750 kg (0.75 tonnes/MW)
Chromium: 400 kg (0.4 tonnes/MW)
Molybdenum: 100 kg (0.1 tonnes/MW)
Zinc: 5,500 kg (5.5. tonnes/MW)
Rare Earths: 50 kg (0.05 tonnes/MW)
TOTAL: 10,100 kg (10.1 tonnes/MW)
Solar (crystalline silicon panels)
Copper: 2,850 kg (2.85 tonnes/MW)
Zinc: 25 kg (0.025 tonnes/MW)
Silicon: 4,000 kg (4 tonnes/MW)
TOTAL: 6,875 kg (6.875 tonnes/MW)
Nuclear
Copper: 1,450 kg (1.45 tonnes/MW)
Nickel: 1,300 kg (1.3 tonnes/MW)
Manganese: 100 kg (0.1 tonnes/MW)
Chromium: 2,200 kg (2.2 tonnes/MW)
Hafnium: 0.5 kg (0.0005 tonnes/MW)
Yttrium: 0.5 kg (0.0005 tonnes/MW)
TOTAL: 5,051 kg (5.051 tonnes/MW)
Elsewhere the IEA 2022 report (pp. 70-72) included mineral calculations for biofuels (biomass), geothermal and hydroelectric plants. One will notice below that geothermal has very high mineral numbers. This is not an error; it is due to the harsh, high temperature and high-salt environments these plants must withstand, requiring large quantities of corrosion- and heat-resistant alloyed stainless steel. No mineral calculations were made for solar thermal plants, so these will be omitted.
Hydroelectric
Copper: 1,050 kg (1.05 tonnes/MW)
Manganese: 200 kg (0.2 tonnes/MW)
Nickel: 30 kg (0.03 tonnes/MW)
TOTAL: 1,253 kg (1.253 tonnes/MW)
Geothermal
Nickel: 118,125 kg (118.125 tonnes/MW)
Chromium: 63,125 kg (63.125 tonnes/MW)
Molybdenum: 6,250 kg (6.25 tonnes/MW)
Titanium: 1,250 kg/MW (1.25 tonnes/MW)
TOTAL: 188,750 kg (188.75 tonnes/MW)
Biofuels:
Copper: 2,270 kg (2.27 tonnes/MW)
Titanium: 400 kg (0.4 tonnes/MW)
TOTAL: 1,125 kg (1.125 tonnes/MW)
While the IEA report’s future projections pertaining renewable power are grossly underestimated (see Michaux, 2021, pp. 653-655 and Table 26.23 above), we can utilise their material ratios above to convert Michaux’ Scenario F power plant estimations to material quantities.
However, to do this we need to convert Michaux’s Scenario F annual terawatt hour calculations for each plant type back to MW (megawatt) installed capacity at the ratios calculated by Michaux in Table 4.4 of his report (column 6):
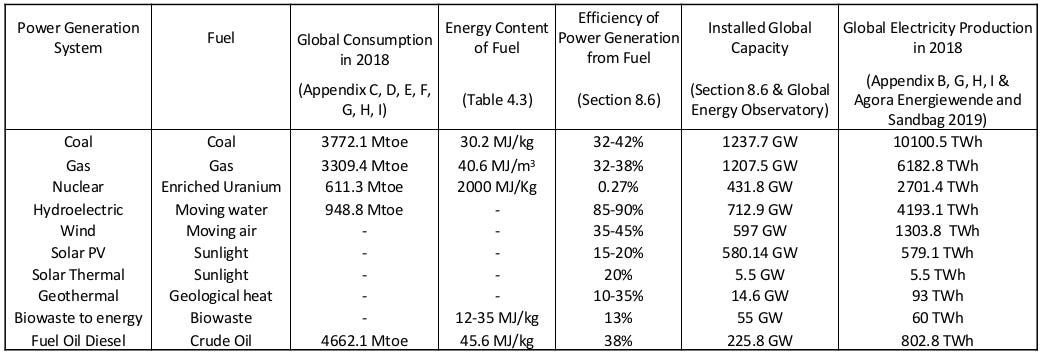
I used Table 4.4 to calculate the average annual number of hours of power generation for each power plant type in 2018 data. Given that there are 8760 hours in a 365-day year, those 2018 calculations were:
Nuclear: 431,800 MW operating 2701.4 TWh = 6256.14 annual hours
Hydro: 712,900 MW operating 4193.1 TWh = 5881.75 annual hours
Wind: 597,000 MW operating 1303.8 TWh = 2183.92 annual hours
Solar PV: 580,140 MW operating 579.1 TWh = 998.21 annual hours
Solar Thermal: (omitted)
Geothermal: 14,600 MW operating 93 TWh = 6369.86 annual hours
Biofuels: 55,000 MW operating 60 TWh = 1090.91 annual hours
Retaining the annual hour ratios for each plant type, we can now do our calculations to convert Michaux’s Scenario F power plants back to MW installed capacity:
834 nuclear plants producing 10,679.7 TWh over 6256.14 annual hours = 1,707,074.97 MW or 2046.85 MW per plant;
12,504 hydroelectric dams producing 16,576.9 TWh over 5881.75 annual hours = 2,818,361.88 MW or 225.4 MW per dam;
63,445 wind farms producing 5,154.4 TWh over 2183.92 annual hours = 2,360,159.7 MW or 37.2 MW per wind farm;
69,291 solar farms producing 2,311.1 TWh over 998.21 annual hours = 2,315,244.28 MW or 33.4 MW per solar farm;
609 geothermal plants producing 367.7 TWh over 6369.86 annual hours = 57,724.97 MW or 94.79 MW per plant; and
74,628 biowaste/biomass/biofuel plants producing 2,580.8 TWh over 1090.91 annual hours = 2,365,731.36 MW or 31.7 MW per plant.
Now, at last (and thank you so much for sticking with me through these laborious calculations), we can combine the IEA and Michaux’s reports to calculate the total mineral quanities required to build Michaux’s 221,594-strong clean energy power plant fleet. Only one final assumption is required: I assume that 70% of the wind farms are offshore, and 30% onshore. We’re finally ready to see how much minerals Michaux’s Scenario F power plant fleet will require to build:
Nuclear (834 plants; 1,707,074.97 MW)
Copper: 2,475,258.7 tonnes
Nickel: 2,219,197.5 tonnes
Manganese: 170,707.5 tonnes
Chromium: 3,755,564.9 tonnes
Hafnium: 853.5 tonnes
Yttrium: 853.5 tonnes
Hydroelectric (12,504 dams; 2,818,361.88 MW)
Copper: 2,959,280 tonnes
Manganese: 563,672.4 tonnes
Nickel: 84,550.8 tonnes
Offshore wind (44,412 offshore wind farms and 1,652,111.79 MW)
Copper: 13,216,894.3 tonnes
Nickel: 495,633.5 tonnes
Manganese: 1,156,478.2 tonnes
Chromium: 826,055.9 tonnes
Molybdenum: 165,211.2 tonnes
Zinc: 9,086,614.8 tonnes
Rare earths: 495,633.5 tonnes
Onshore wind (19,033 onshore wind farms and 708,047.7 MW):
Copper: 2,053,338.3 tonnes
Nickel: 283,219.1 tonnes
Manganese: 531,035.8 tonnes
Chromium: 283,219.1 tonnes
Molybdenum: 70,804.8 tonnes
Zinc: 3,894,262.4 tonnes
Rare Earths: 35,402.3 tonnes
Solar (69,291 solar farms and 2,315,244.28 MW)
Copper: 6,598,446.2 tonnes
Zinc: 57,881.1 tonnes
Silicon: 9,260,977.1 tonnes
Geothermal (609 plants and 57,724.97 MW)
Nickel: 6,818,762.1 tonnes
Chromium: 3,643,888.7 tonnes
Molybdenum: 360,781.1 tonnes
Titanium: 72,156.2 tonnes
Biofuels (74,628 plants and 2,365,731.36 MW)
Copper: 5,370,210.2 tonnes
Titanium: 946,292.5 tonnes
And our grand mineral totals to build Michaux’s 221,594 power plants for a clean energy future?
Copper: 32,673,427.5 tonnes
Nickel: 9,901,393 tonnes
Manganese: 2,421,893.9 tonnes
Zinc: 13,038,758.3 tonnes
Chromium: 8,508,728.6 tonnes
Molybdenum: 596,797.1 tonnes
Silicon: 9,260,977.1 tonnes
Titanium: 1,018,448.7 tonnes
Rare Earths (including Hafnium & Yttrium): 532,742.8 tonnes
This analysis could not possibly be completed without noting the 2022 production rates for each mineral and the time it would take to amass the quantities listed above, assuming 100% of the required metals were diverted away from other industries:
Copper: 26 million tonnes of refined/recycled copper per year (2022 data), with 5.6 billion tonnes of potential reserves.9 Thus, it would take 1 year and 3 months to amass the necessary copper.
Nickel: 3.3 million tonnes from mining per year (2022 data), with 300 million tonnes of potential reserves.10 Thus, it would take 3 years to amass the necessary nickel.
Manganese: 1 million tonnes of refined metal per year (2021 data),11 with 1.7 billion tonnes of potential reserves (2022 data).12 Thus, it would take 2 years and 5 months to amass the necessary manganese.
Zinc: 13.49 million tonnes of refined metal per year (2022 data), with 1.9 billion tonnes of reserves.13 Thus, it would take less than 1 year to amass the necessary zinc.
Chromium: 41 million tonnes of chromium oxides suitable for steel applications per year, with 12 billion tonnes of potential reserves.14 Chromium is thus a metal of least concern in this scenario, as it would take less than 2 months to amass the necessary amount of chromium.
Molybdenum: 250,000 tonnes per year (2022 data), with 20 million tonnes of potential reserves.15 Thus, it would require 2 years and 5 months to amass the necessary amount of molybdenum.
Silicon: 8.8 million tonnes per year (2022 data), with unquantified potential reserves.16 Silicon is thus a material of least concern in this scenario; amassing the required amount would take just over a year. Manufacturing it into silicon crystal suitable for solar PVs is another matter, however.
Titanium: 260,000 tonnes per year (2022 data) and maximum capacity of 350,000 tonnes per year, with potential reserves estimated at 700 million tonnes.17 At maximum capacity, it would take just under 3 years to amass the necessary titanium.
Rare Earths: 300,000 tonnes per year (2022 data), with 130 million tonnes of potential reserves.18 It would thus take 1 year and 9 months to amass the necessary rare earths (especially neodymium, dysprosium, praseodymium and terbium).
In a final twist, it turns out that, suspending all other industrial applications for 1-3 years, there are indeed enough critical minerals to build Michaux’s 221,594 power plant clean dream world. At least until the solar and wind installations need replacing every 20 years or so. Oops. Yeah, the maintenance issue rears its ugly head once more.
Series Conclusion
It feels nice to conclude on a high that humanity’s energy situation is not all doom and gloom. We saw above that, despite the difficulties transitioning the world’s vehicle fleets to EVs, there actually are enough minerals to build Michaux’s 221,594 power plants to transition the world away from coal, oil and gas. Perhaps a 100% hydrogen economy is actually the better answer after all. What manner of environmental devastation will accompany such mass mining, mass refining and mass manufacturing endeavours remains to be seen. I cannot imagine that the world’s bird and bat life will appreciate 63,445 new 37 MW wind farms dotted across sea and land. Neither am I confident that it will be a smooth ride in the decades ahead. There is a lot of money to be made in the clean energy transition, after all.
Michaux’s report ultimately concluded with six key challenges for the clean transition agenda (pp. 662-665) which are worth repeating here:
Not enough time to meet construction targets;
Sourcing enough minerals to supply manufacture of renewable technology;
Developing enough power storage to mitigate intermittent power supply;
Finding enough suitable sites for hydroelectric power plants;
Phasing out petrochemical fertilizers, herbicides, and pesticides;
Human population growth.
These challenges are where we should be placing the emphasis of our time, debate and investment dollars for innovative solutions. As it stands, global think-tanks like the WEF, IEA and national governments have idealised 2030 and 2050 visions without actually crunching real numbers involved. These transition projects are much larger in scale than they envision.
As I mentioned in my conclusion to Part 2, we ought to be having discussions about phasing out chemical fertilisers, herbicides and pesticides. Those mammoth industries with do everything in their power to prevent the abolition of their destructive, cancer-causing products, so we cannot expect them to fade quietly into the non-fossil-fuel wind. This will most certainly require a shift away from mass industrial agriculture and a return to small-hold, organic, regenerative agricultural enterprise of our forebears. We need to have those conversations as to whether we would voluntarily become the peons of a looming global technocracy. At the moment I am not confident in our ability to persuade governments and industrial-scale farmers to allow us to return to older, simpler ways of life, or redistribute ‘crown’ land for such purposes. Neither am I confident that the powers-that-be will not just pull the pin on challenge no. 6 and wipe the planet clean for themselves and their AI-cyborg-hive mind progeny, or whatever ridiculous transhumanist plots they devise in secret.
I started this series as a scoffing climate-sceptic. I still am one. I do not believe CO2 emissions cause climate change (the earth’s interconnected plasma-electric relationship with the sun and wider galaxy [cosmic rays] are the primary driving forces of earth weather). None of that was under discussion in this 4 part series. We only sought to answer Michaux’s clean transition question, “Can it be done?” We answered with a resounding, “It cannot be done and will not be done.”
However, I am now a wholly-persuaded energy crisis advocate. One day in the not-too-distant future, coal, oil and gas will diminish lower that sweet spot of 11:1 ERoEI (see Part 2 for the ERoEI discussion). If new, equivalent ERoEI systems of energy production are not in place by then, modern western civilisation will commence its decline to complete energy destabilisation. This may not occur for another 100-200 years. But we do need to have conversations now regarding what we will do about it. We cannot afford to let globalist technocrats hold the only sway in the debate. We cannot afford to be an idle, TV-absorbing, consumerist culture any more. It is time for a new form of universal iconoclasm: the idols of capitalism, infinite growth and unlimited production and consumption need to be smashed to pieces on the ground. This is the kind of societal restructure I think Michaux hinted at in his conclusion (p. 669). And we need to drag the globalist parasite class down to reality, kicking and screaming, with us.
One final thing. If you want to make some money on the transition-critical minerals and the idiots who will likely run full steam ahead in this ideological agenda, this is what you should consider betting your money on:
It’s been a fun series. See you soon for my next Full Broadside against the world of viruses and virology.
I cannot help but make a suggestion here: bring back the zeppelin! Solar/Hydrogen fuel cell zeppelins might be able to fill a new role in ‘clean energy’ future aviation. They have surface area for solar panels and volume for hydrogen gas. One of the big issues with airships of the early 20th century was venting or compressing gas (either hydrogen or helium) for landing; this could be done by using H2 gas as fuel in a hydrogen fuel cell system for the landing leg of a journey.
Jan Martin, Katharina Schafner, and Thomas Turek (2020), “Preparation of Electrolyte for Vanadium Redox-Flow Batteries Based on Vanadium Pentoxide.” Energy Technology 8 no. 9: 2000522, p. 1. DOI: 10.1002/ente.202000522
See Table 2 in Jens Noack et al. (2016), “Techno-Economic Modeling and Analysis of Redox Flow Battery Systems.” Energies 9 no. 8: 627. DOI: 10.3390/en9080627
Christine Minke, Ulrich Kunz and Thomas Turek (2017b), “Techno-economic assessment of novel vanadium redox flow batteries with large-area cells.” Journal of Power Sources 361: 105-114. DOI: 10.1016/j.jpowsour.2017.06.066
For details on VRFB membranes and their development, see Birgit Schwenzer et al. (2020), “Preparation of Electrolyte for Vanadium Redox-Flow Batteries Based on Vanadium Pentoxide.” Energy Technology 8 no. 9: 2000522 DOI: 10.1002/ente.202000522; and Helen Prifti et al. (2012), “Membranes for Redox Flow Battery Applications.” Membranes 2 no. 2: 275-306 DOI: 10.3390/membranes2020275.
For details on SPEEK membranes, see Suminto Winardi (2014), “Sulfonated poly (ether ether ketone)-based proton exchange membranes for vanadium redox battery applications.” Journal of Membrane Science 450: 313-322. DOI: 10.1016/j.memsci.2013.09.024
For details on carbon-felt electrodes, see Christine Minke, Ulrich Kunz and Thomas Turek (2017a), “Carbon felt and carbon fiber - A techno-economic assessment of felt electrodes for redox flow battery applications.” Journal of Power Sources 342: 116-124. DOI: 10.1016/j.jpowsour.2016.12.039
For details on carbon-polymer bipolar plates, see Christine Minke et al. (2016), “Cost and performance prospects for composite bipolar plates in fuel cells and redox flow batteries.” Journal of Power Sources 305: 182-190. DOI: 10.1016/j.jpowsour.2015.11.052
USGS (2023), “Nickel.”
USGS (2023), “Manganese.”
USGS (2023), “Zinc.”
USGS (2023), “Chromium.”
USGS (2023), “Molybdenum.”
USGS (2023), “Silicon.”
USGS (2023), “Titanium” and “Titanium Mineral Concentrates.”
USGS (2023), “Rare Earths.”
POSTSCRIPT
I received some excellent, albeit parochial, criticism of Michaux's nuclear Scenario E by SmithFS which I want to include in the debate for balance:
https://celiafarber.substack.com/p/green-tyranny-by-rupert-darwall/comment/14816670#comment-14819119
I asked SmithFS if he would redo Michaux's Scenario E with his own nuclear picture. We will see if that eventuates into a more positive picture for humanity’s energy future.
I confess I only skimmed through this, apologies, you've put in a lot of work. My two bobs:
1) There was a uranium dude on the recent decouple podcast who reckons there is plenty of uranium around
2. None of the people who make decisions or even MSM are doing this kind of analysis, or , probably because they don't have the ability to read more than an A3 page with a couple of charts on it. But they really ought to.
3. And even if Uranium runs out in 2095, I personally don't give a shit. Every other doomsday prediction has not come to fruition, so I'm basically not planning more than 5 - 10 years ahead with respect to my own life. AFAIK, Al Gore's prediction that the arctic was going to melt by 2022 hasn't come true.