"Clean Energy Transition" Part 3
Reassessing the global feasibility of converting the world's transportation to electric-battery, hydrogen fuel cells and/or biofuels.
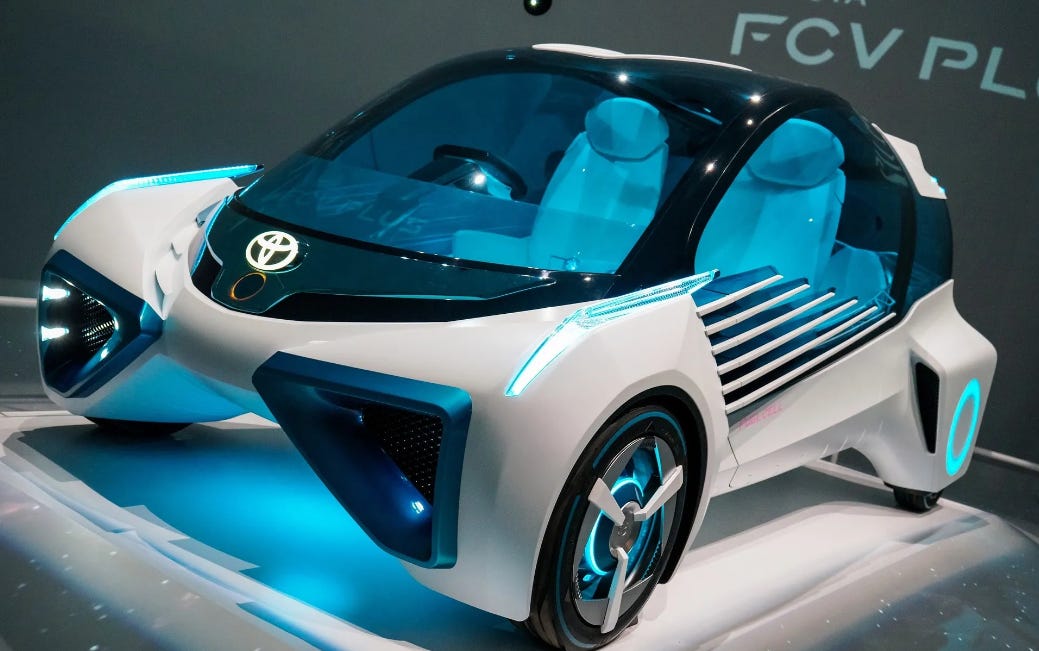
We now arrive at our third installment in this “Clean Energy Transition” series. Part one detailed the absurdly expensive and logistically impossible task for Australia alone to transition to CO2-free, renewable energies. Part two took the task global, uncovering that an identical civil engineering nightmare applied to any global desire for a clean, non-fossil fuel energy transition by 2050: 181 (33 GWh capacity) solar farms; 20 (80 GWh) geothermal, tidal or biowaste plants; 164 (81 GWh) wind farms; 32 (1325 GWh) hydroelectric dams; and 2 (12,800 GWh) nuclear power plants must be constructed per month, every month, from 1 Jan. 2022 through 31 December 2050. It cannot be done and will not be done.
We now return our attention to Simon Michaux’s August 2021 report, “Assessment of the Extra Capacity Required of Alternative Energy Electrical Power Systems to Completely Replace Fossil Fuels,” to explore a hypothetical transition of the entirety of the world’s transportation infrastructure away from oil and gas to clean renewables. Taking the lead from Michaux, we will be exploring his Scenario A (replace all combustion engines with EVs), Scenario C (replace all combustion engines with hydrogen fuel cells) and Scenario D (replace fossil fuels with biofuels).
Clean Energy Benchmarks: World Combustion Engine Fleets
Before we can embark on those three scenario thought-worlds, we must do as we have done in every other article of this series: establish the necessary energy benchmarks. In this case, we need to know the size of the world’s combustion engine fleets which are destined for electrical transition. Michaux details this at length in chapters 12-15 (pp. 265-309) of his report (with reference to his relevant appendices, particularly Appendices J, K, N & O). Briefly summarised, the estimated world transportation fleet consists of:
1,416,528,615 road vehicles (all classes) travelling 15.87 trillion kms per year.
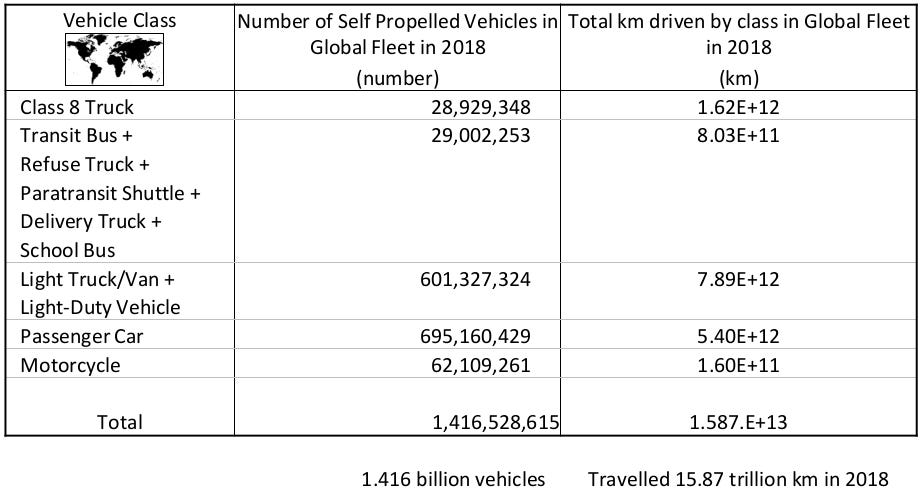
104,894 diesel freight trains (85% of the total rail freight fleet) hauling 12,545,000,000 tonnes of goods over 11,067,000,000,000 kms per year, and accounting for 45% of passenger rail transport (Michaux, 2021, Appendix K, pp. 810-812 and 815).
Electric trains (no number given) consuming a combined approximate of 290 terawatt hours of power annually to transit 32,355,000,000 passenger journeys (55% of the global total) over 3,823,000,000,000 kms per year, and account for 15% of rail freight transport (Michaux, 2021, Appendix K, pp. 813-814).
116,857 ships of all sizes and categories hauling a combined 11,005,000,000 tonnes of goods an average journey length of 8832 km (Michaux, 2021, Appendix N, pp. 891-902).

30,379 aircraft of all sizes greater than 9 tonnes transporting 4,377,670,000 passengers and 62,487,000 tonnes of goods 8,329,776,000,000 kms per year (Michaux, 2021, Appendix O, pp. 903-911).
Those are some pretty impressive numbers to set our clean energy transition benchmarks for the transportation sector. Now, let’s play with these scenarios and see what the world needs to do to transition transportation away from oil to something else.
Summary: 100% EV vs 100% Hydrogen Global Transportation Fleet
For those whose time is limited, the direction we are going in this article is as follows, remembering we already covered Scenario B (fossil fuel power to be phased out) in Part 2:
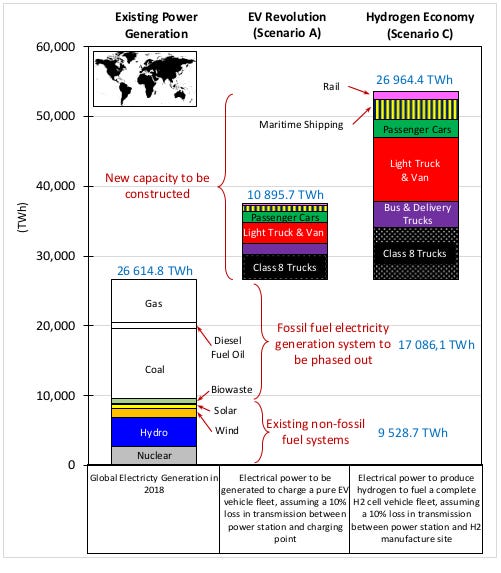
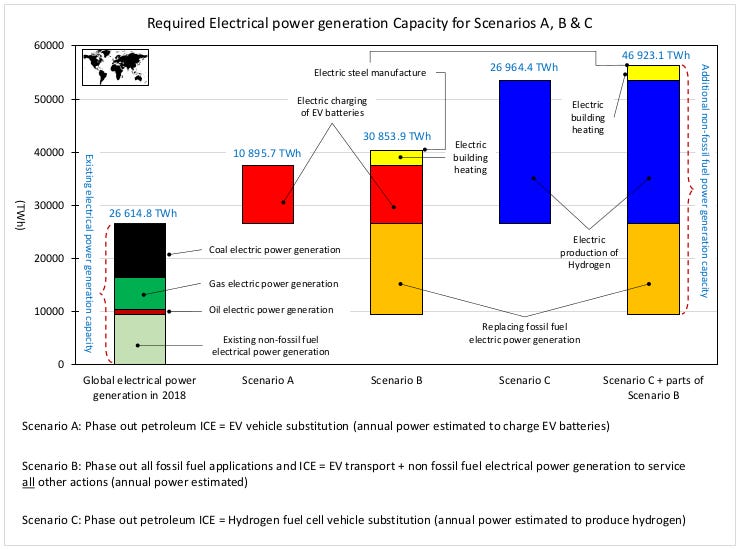
Scenario A: Everything’s Electric!
In this thought experiment (chapters 16-18, pp. 310-381), Michaux transitioned the global transportation fleet to electric via lithium-ion (NMC 811 - Nickel 80%, Manganese 10%, Cobalt 10% cathode) batteries, the most energy-dense battery currently available in widespread use. Included in his calculations was required extra grid capacity to power the world’s electric fleets due to the fact that, though more efficient than internal combustion engines, electric motors relying on battery power are operating at 55 times lower energy density than combustion fuels.
The total extra electrical capacity required to power a 100% EV transportation fleet was an additional 10,895.2 terawatt hours of power. This is on top of the 9528.7 terawatt hours of existing carbon-zero electrical power and 17,086.1 terawatt hours of global fossil fuel power destined for transition to renewables:
9,722.7 terawatt hours for 100% EV road vehicles;
226.6 terawatt hours for 100% EV rail;
945.9 terawatt hours for 100% EV ships;
Planes could not be reasonably transitioned to electric due to battery weights interfering with take-off plausibility.
Included in this calculation was the mass of lithium-ion (NMC 811) batteries that must be manufactured to effect the transition away from oil and gas combustion engines in the global transportation fleet: 870 million tonnes of NMC 811 lithium-ion batteries will be required.
339 million tonnes for EV road vehicles;
29.5 million tonnes for EV trains;
450.5 million tonnes for EV ships;
51.3 million tonnes as static base-load power to provide a 48 hr (11.8 terawatt hour) buffer for the intermittent nature of solar and wind renewables.
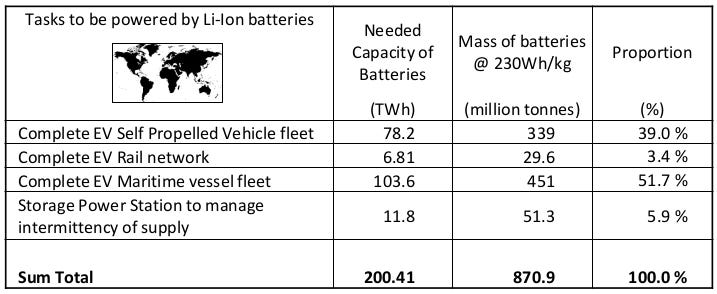
We will return to the acquisition of the criticial minerals for these batteries in Part 4. Below I include Michaux’s tables and numbers for the calculations of each transportation class.
Road
Michaux divided road vehicles by class, totalled the kilometers driven per class, and then converted their energy needs to lithium-ion battery equivalents in the following table. The total extra electrical capacity to power a 100% EV road fleet, globally, would be approximately 9,722.7 terawatt hours annually (click to enlarge image).
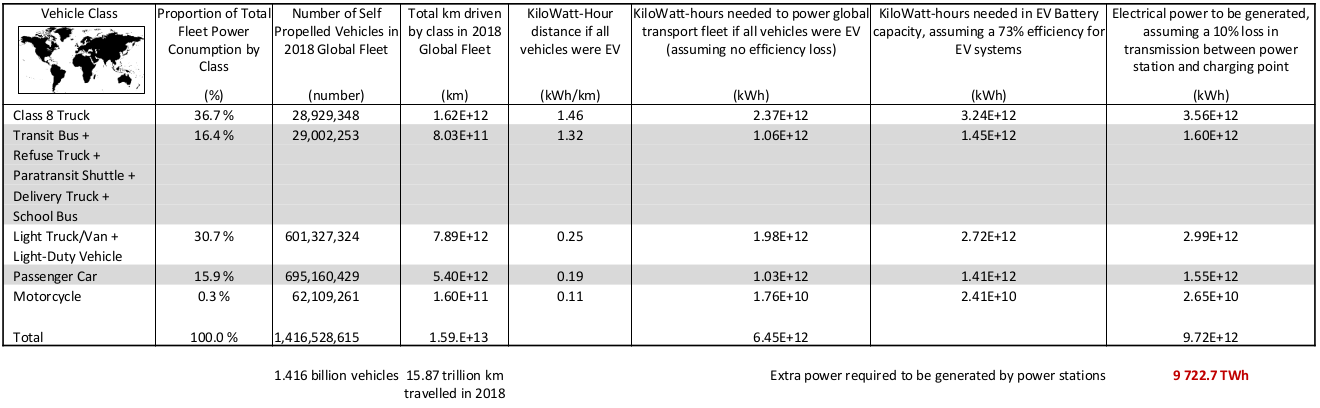
The total number of lithium-ion batteries to be manufactured was also calculated: 339 million tonnes will be required to transition combustion engine road vehicles to electric in this scenario:
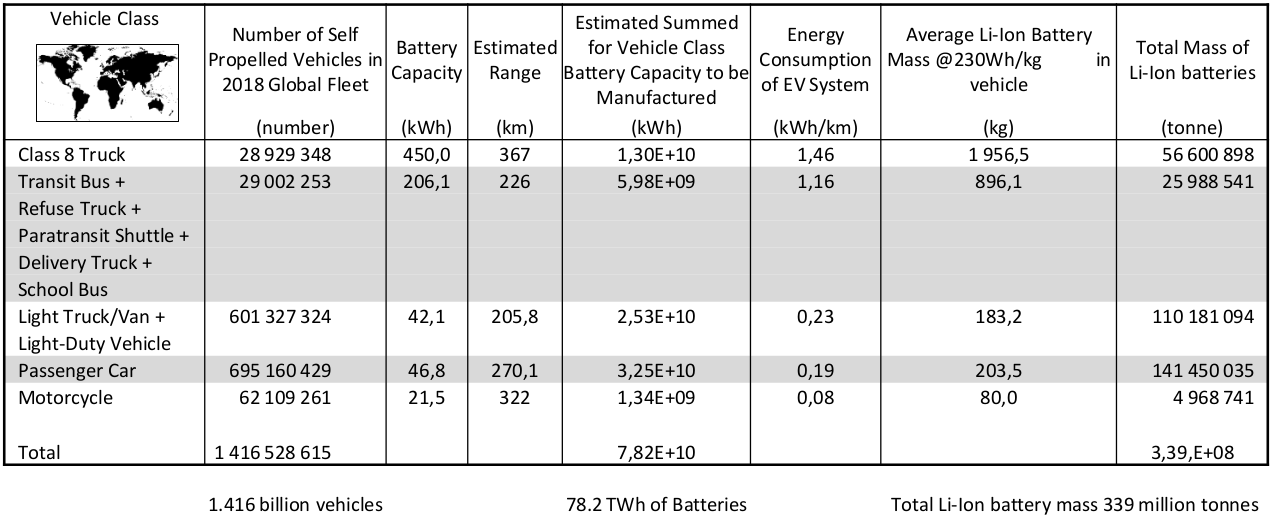
We will return to the feasibility of mining the critical minerals for these 339 million tonnes of batteries in Part 4.
Rail
The rail task was to convert the 45% of diesel rail passenger journeys and 85% of diesel rail freight journeys to renewable electric. Michaux’s overall estimate (pp. 311-312) was an additional 226.6 terawatt hours of electricity will be required to power an electrical transition of the global diesel rail fleet in addition to the 290 terawatt hours already powering electric trains globally. Not included in this calculation was the fact that many current diesel-only long distance rail lines, such as Trans-Siberian, Indian-Pacific and Pan-American, would also require electrification infrastructure upgrades (i.e., poles and wires).
Like with road transport, an electrified rail system may be impractical in all situations. Thus, an electrified, formerly-diesel passenger-freight rail network utilising an electric engine equivalent to a GE Transportation AC6000CW (6,000 hp/ 4,500 kW) may require the use of batteries for non-electrified portions of a route. Michaux includes those calculations as well (p. 313): an electric freight train hauling 3,000 tonnes of goods 800 km (500 mi) would require a lithium-ion (NMC 811) battery bank weighing 282 tonnes (65,000 kWh) to replace what would otherwise be 12,000 litres (approx. 12 tonnes) of diesel fuel. Transitioning the world’s 104,000 diesel freight trains to electric-battery would require at least 29.5 million tonnes of batteries. We will return to the feasibility of mining and manufacturing that many batteries in Part 4.
Ship
The majority of world freight is transported by ship, so electrifying this almost exclusively marine diesel fleet would be a necessity to achieve Net Zero targets. The world’s 116,857 ships have varying sizes (gross tonnage) which complicates transition calculations. Small ships (100-499 gross tonnes) account for 46% of the global fleet, but carry only 1% of global freight; megafreighters (greater than 60,000 gross tonnes) account for a mere 6% of the global fleet, but carry 49% of the world’s freight:
Each class also has its own fuel consumption needs depending on sailing speed. Michaux used an average speed of 20 knots; each ship class’s fuel consumption thus ranged from 8.6 tonnes/day (see column 5 in Table 17.1 above) for small ships, 27 tonnes/day for medium, 75 tonnes/day for large and 175+ tonnes of marine diesel per day for megafreighters. The total marine fuel and energy requirements to ship to various world ports for each ship class was calculated as follows:
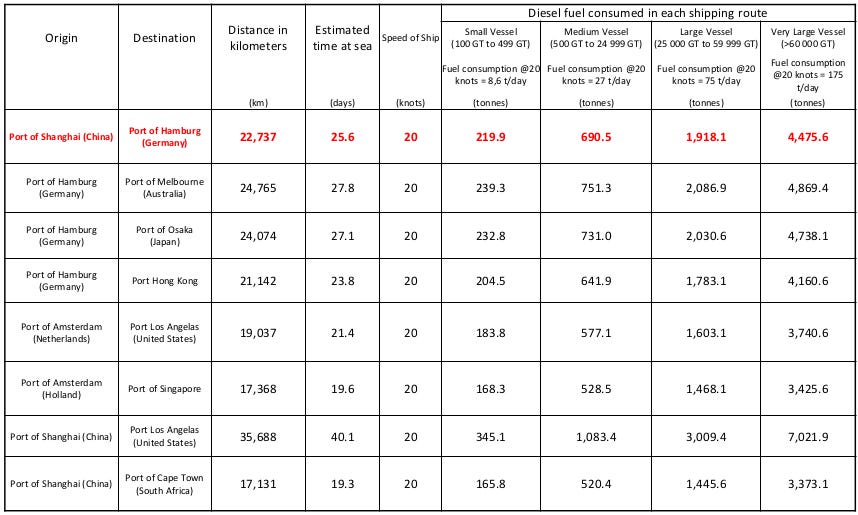
With 38% fuel efficiency in marine diesel engines and fuel energy of 12,750 Wh/kg, the total energy in kilowatts (kW) for each shipping journey can be calculated:
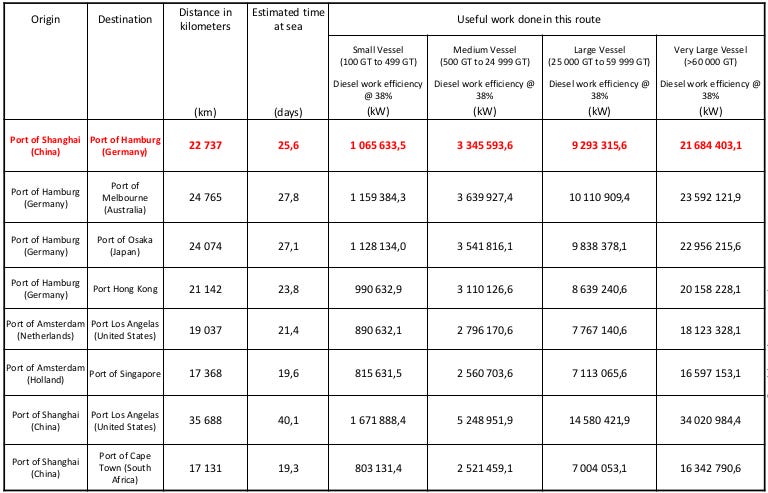
We are now ready to covert across to 73% efficient, 230 Wh/kg lithium-ion (NMC 811) battery propulsion systems for those same journeys:
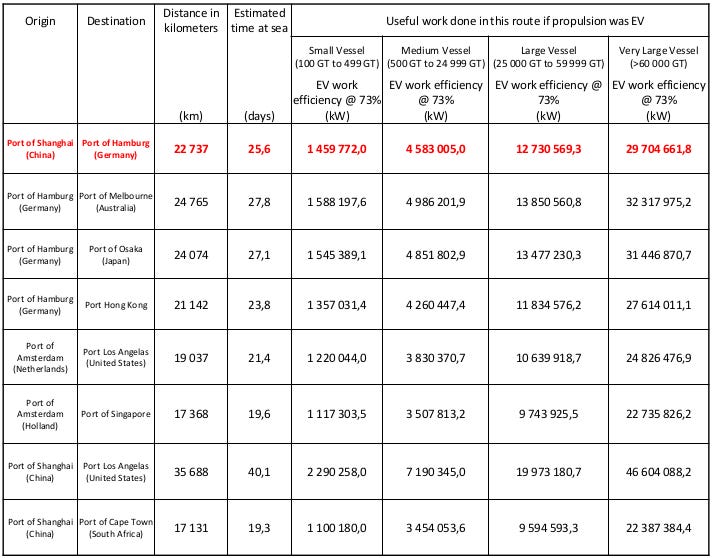
And last of all, the mass of lithium-ion (NMC 811) batteries for journeys of representative lengths were tabulated and compared to necessary reductions in cargo space for the various ship size classes:
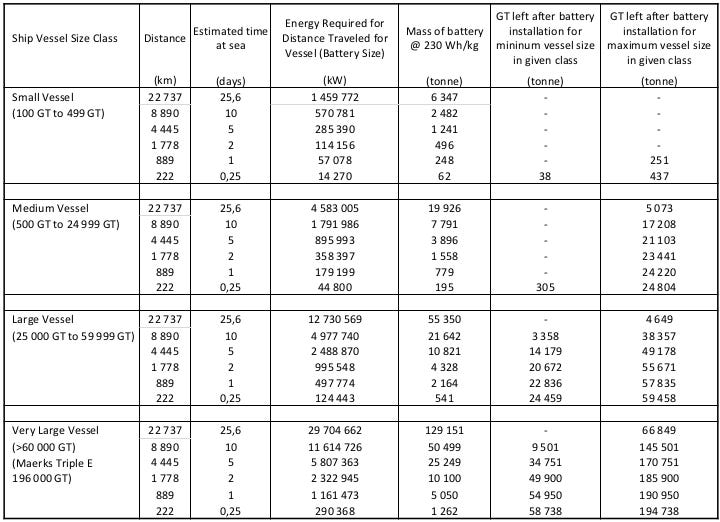
One of the huge issues that develops for any conceptual transition of large engines like world shipping, as seen from the above Table 17.15, is that marine fuel oil has an energy density of 12,750 Wh/kg and energy efficiency of 38%, whereas the most advanced lithium-ion (NMC 811) batteries have a mere 230 Wh/kg and 73% efficient electric motors. Any proposal to convert fuel oil shipping to batteries is going to result in significant cargo space losses to those batteries because a lithium-ion battery bank is always going to occupy a larger volume than several thousand tonnes of marine fuel oil.
Note some of the numbers here (Table 17.15)! A very large megafreighter, the type often used for long routes, e.g. Shanghai to Hamburg (22,737 km), would not be able to fit a single battery for that whole journey as the battery alone would weigh 129,151 tonnes. An 8890 km range battery for a megafreighter would weigh 50,499 tonnes, thus reducing cargo capacity by 74.2%, and it must recharge twice to undertake a journey like Shanghai to Hamburg. Thus, the entire marine fleet requires a restructure (Michaux, 2021, p. 333):
To convert the maritime cargo fleet to EV, it is practical to have all shipping transport conducted by Large and Very Large vessels, where Very Large is estimated using the Maersk Triple E-class container ship. Smaller ships (Medium and Small) would be tasked to transport of people and cars over short distances. To make this adjustment, the Very Large vessel proportion of 49% gross tonnage from Table 17.20 becomes 59.8% of global cargo commodity transport. The Large vessel proportion of 33% from Table 17.20 becomes 40.2% of global cargo commodity transport.
The final calculations can now be made of this hypothetically restructured global shipping fleet:
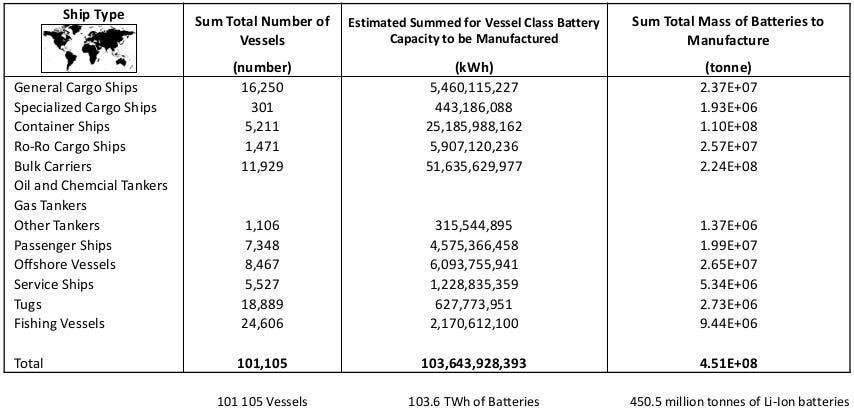
Read those numbers again. 101,105 vessels (down from the earlier 116,857 due to the necessary fleet restructure) carrying 450.5 million tonnes of lithium-ion batteries which themselves require 945.9 terawatt hours of electricity to charge (Michaux, 2021, p. 335).
Plane
Michaux (p. 355) assessed aircraft EV transitions as entirely implausible:
The EV solution for aviation was considered not viable due to the mass requirements of the batteries, which would be far too heavy to be practical.
Aircraft are thus excluded from the 100% EV scenario.
Total New Power Plants
An additional 10,895.2 terawatt hours of power (on top of the 9,528.7 terawatt hours of power produced globally by the world’s non-fossil fuel power plants) is required to transition the world’s transportation fleets to EV:
9,722.7 terawatt hours for 100% EV road vehicles;
226.6 terawatt hours for 100% EV rail;
945.9 terawatt hours for 100% EV ships.
This is where a portion of Michaux’s figures mentioned in Part 2 came from. To transition the world’s transportation fleets to 100% electric-battery will inherently require the construction of an additional 64,093 non-fossil fuel power plants. At 2018 global proportions, this would mean an additional:
241 (12,800 GWh) nuclear power plants;
3,617 (1325 GWh) hydroelectric dams;
18,351 (81 GWh) wind farms;
20,123 (33 GWh) solar farms;
21,761 (80 GWh) geothermal, tidal or biofuel plants.
Again, these additional power plants are only to power a 100% EV global transportation fleet and excludes the transitions of heavy industry like refining, smelting and mass manufacturing (those transitions were included in Part 2, Table 23.10, an additional 19,958 terawatt hours of annual fossil fuel energy consumed by heavy industry).
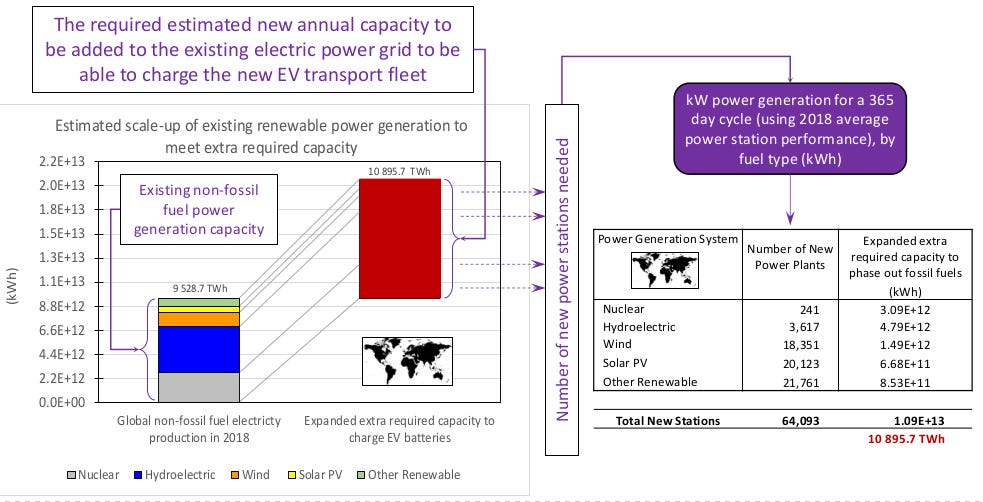
If there is any one point that emerges from the 100% EV transition, as Michaux himself remarked (p. 380), it is the fact that long-range transportation systems such as freight rail and long-haul shipping need an alternative power system to lithium-ion (NMC 811) batteries!
Scenario B: Hydrogen will Help!
One renewable, CO2-free power source identified by Michaux (chapters 19-20, pp. 382-426) for such long-range applications is hydrogen fuel cell technology which utilises reverse electrolysis to generate electricity from hydrogen and oxygen.
Michaux (p. 399) limited his analysis to Polymer Electrolyte Membrane cell electrolysers (PEM fuel cells) on the simple fact they are the most well-proven and industrially-applicable. Two other types, Phosphoric Acid (PC) and Molten Carbonate (MC) fuel cells, do exist, but they operate at much higher temperatures than PEM cells.
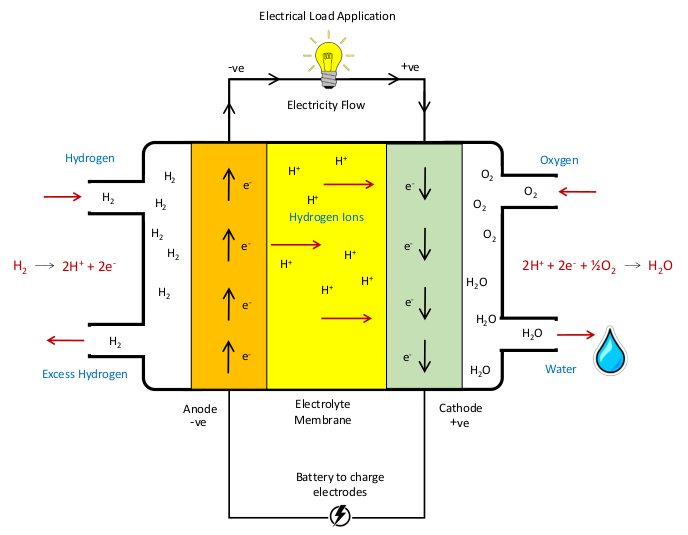
The first problem that arises, however, is that the majority of the world’s hydrogen is sourced from fossil fuels!
Globally, 73.9 million tonnes of hydrogen are produced from all of the above sources (as of 2018 data). Only 3 million tonnes of hydrogen is produced by electrolysis.
Since coal, oil and gas are supposed to be phased out entirely in these Net Zero by 2050 models, only electrolysis remains as a viable clean transition option to obtain hydrogen as fuel. Michaux’s estimate (Figure 19.27, below) was 50 kWh of electricity is required to electrolyse 10 kg of water into 1 kg of hydrogen (with 9 kg oxygen as a by-product). One kilogram of hydrogen can produce 15 kWh of electricity in a PEM fuel cell vehicle with a byproduct of 15 kWh of heat (and water as waste).
The second problem is how to store and/or transport hydrogen safely. As everyone knows from the 1937 Hindenburg zeppelin disaster, hydrogen is an extremely flammable gas which reacts explosively with oxygen in the air. What is lesser known is that it is also extremely permeable, that is, it can escape containment by passing through the tiniest cracks, and it severely corrodes metal piping. There are three viable options for hydrogen transport: liquid, compressed gas or metal hydrides. The liquid option obtains the highest hydrogen fuel density of 70.8 kg/㎥, but is severely limited by hydrogen’s liquification temperature of -252.8 °C. Thus, liquid hydrogen requires special cryogenic storage containers. As a compressed gas, its fuel density depends entirely on its pressure. Michaux (p. 397) used the figure of 700 bar (70,000 kPa or 10,000 Psi), at which pressure hydrogen has a fuel density of about 40 kg/㎥. It requires between 2.7-13.5 kWh of energy to compress hydrogen to this pressure, but Michaux used 2.5 kWh as his figure, expecting future advances in hydrogen pressurisation to improve efficiency in this area. Metal hydrides are an emerging technology whereby metal alloys are used like a chemical sponge to absorb hydrogen atoms, which can be released at a later time under heating or a specific catalyst added to the fuel tank. These are not yet suited to any industrial scale use, however, so they were excluded from Michaux’s analysis.
Overall, this is the estimation for a future hydrogen fuel cell economy:
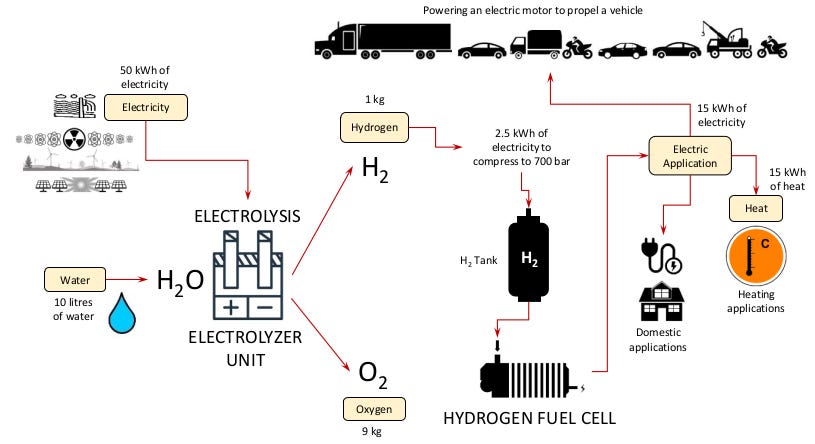
Michaux assumed an average energy efficiency of 50% for the entire process, which was actually more generous than his calculation:
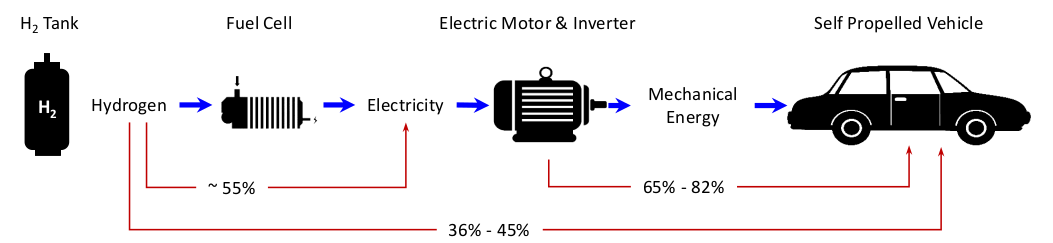
We can now review the numbers crunched by Michaux for a global hydrogen fleet (p. 415). Overall, a global hydrogen transportation fleet will require 502.6 million tonnes of hydrogen production per annum (remembering that 2018 global production was 73.9 million tonnes, of which 70.9 million tonnes comes from coal, oil and gas sources which must also be phased out):
35.7 million tonnes for existing industrial applications which needs to be transitioned away from coal, oil and gas hydrogen sources to 100% electrolysis;
396.8 million tonnes for the global road vehicle fleet;
18.5 million tonnes for the global freight train fleet;
51.66 million tonnes for the global shipping fleet
Aviation cannot be transitioned to hydrogen fuel cells with current technology.
Assuming it takes 50 kWh to electrolyse 1 kg of hydrogen from 10 kg of water, and an additional 2.5 kWh to compress that hydrogen into 700 bar storage tanks, both very generous assumptions well ahead of current estimates, the global energy grid will require an additional 26,964.4 terawatt hours of non-fossil fuel electrical production to transition to a 100% hydrogen fuel cell fleet.
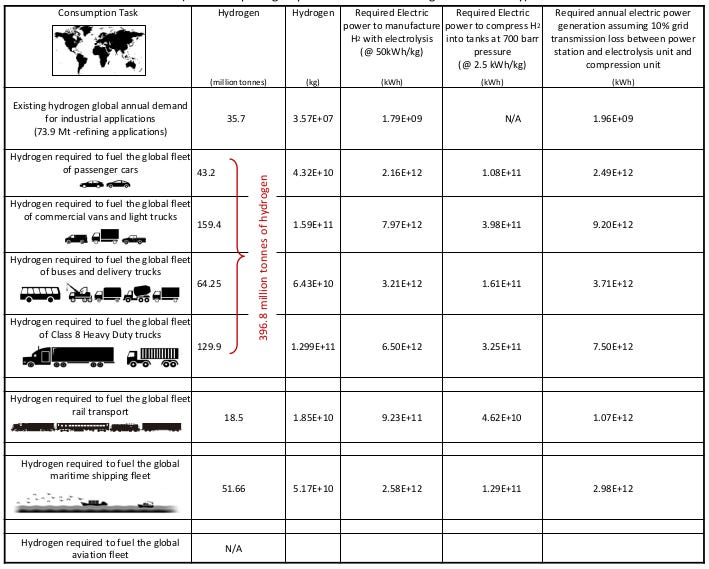
The particulars are below for each transport category.
Road
Using the same numbers as the road fleet calculations above, Michaux’s estimate was 396.8 million tonnes of hydrogen per annum will be required by the global road fleet’s 1.416 billion vehicles if they were transitioned to hydrogen fuel cell technology.
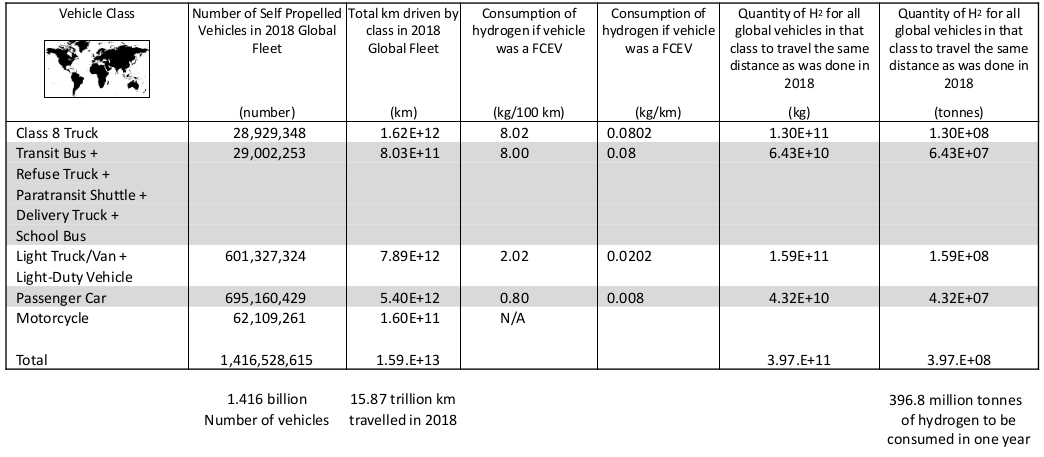
The electrolysis and compression of the hydrogen for the global road fleet will require an additional 22,900 terawatt hours of new electrical energy production annually.
Rail
The 227 terawatt hours of electrical energy required to transition the world’s diesel train fleet to hydrogen fuel cells would require 15.13 million tonnes of hydrogen every year. The electrolysis and compression of that hydrogen will require an additional 1,070 terawatt hours of new electrical energy production annually.
Michaux (p. 410) also helpfully compared hydrogen fuel cell trains to EV trains and the results were stark. Using the same freight metric as above (3,000 tonnes of goods being hauled 800 km/500 mi), a hydrogen fuel cell train would require 4.32 tonnes of hydrogen fuel. An EV train performing the same task would require 282 tonnes of lithium-ion batteries. Clearly, hydrogen fuel cells play a role in supplying the fuel for a clean, long-haul global freight train fleet.
Ship
Michaux estimated (p. 411) that the restructured maritime shipping fleet (as calculated for EV shipping above) would require 51.66 million tonnes of hydrogen fuel every year. This will simultaneously need an additional 2,980 terawatt hours of new electrical energy production annually.
The comparison between fuel cell and EV shipping was similarly stark. A megafreighter travelling 8890 km would require a 50,499 tonne lithium-ion battery, a 13,585.4 tonne compressed hydrogen gas tank, or a 5,531.8 tonne liquid hydrogen cryotank.
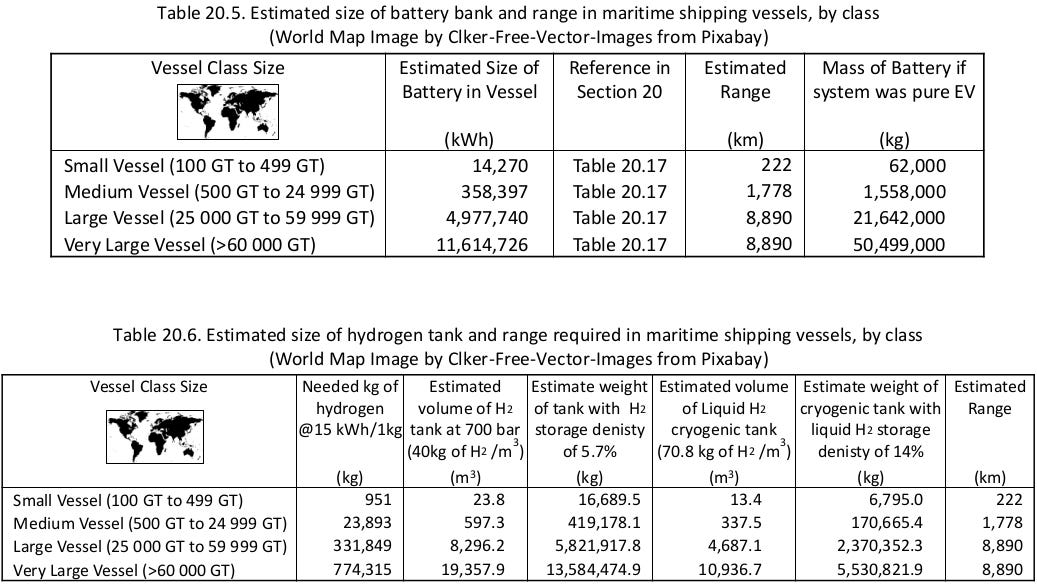
Plane
Once again, no feasible hydrogen fuel cell concepts exist for aircraft (Michaux, 2021, p. 413). The global aircraft fleet cannot be transitioned to hydrogen fuel cells without significant advances and research in aircraft hydrogen fuel cell design and efficiency.
Total New Power Plants
Once again, Michaux included the creation of the required number of new power plants to address this new demand by utilising 2018 world proportionality. To supply the world’s hydrogen fuel cell energy needs, the global power grid requires 158,615 new non-fossil fuel power plants constructed:
597 nuclear power plants;
8,950 hydroelectric dams;
45,414 wind farms;
49,598 solar farms;
202 solar thermal farms;
436 geothermal plants;
53,418 biowaste/biofuel plants.
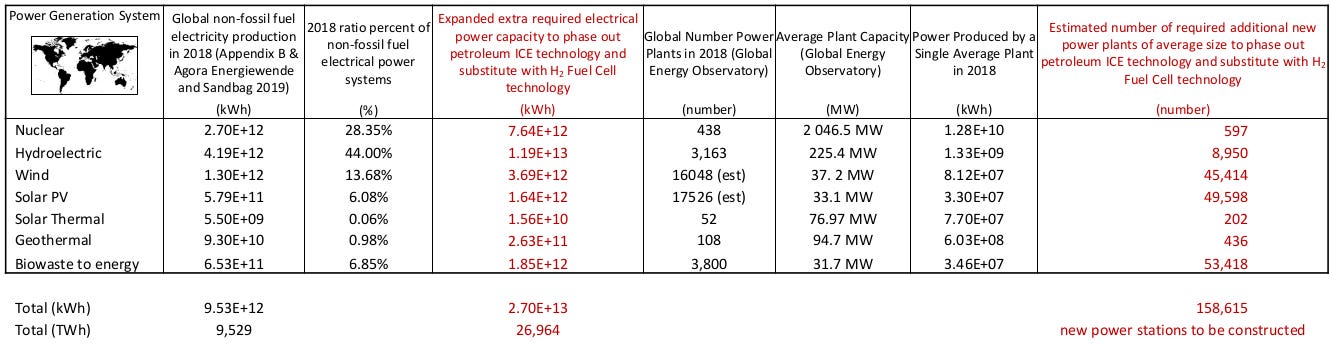
Including the electrical requirements to transition global industry’s 19,958 terawatt hours of coal and gas consumption to non-fossil fuels as well, the task gets even greater: 46,293 terawatt hours of new electrical production from 276,020 non-fossil fuel power plants.
1,039 nuclear power plants;
15,575 hydroelectric dams;
79,029 wind farms;
86,309 solar farms;
352 solar thermal farms;
759 geothermal plants;
92,958 biowaste/biofuel plants.
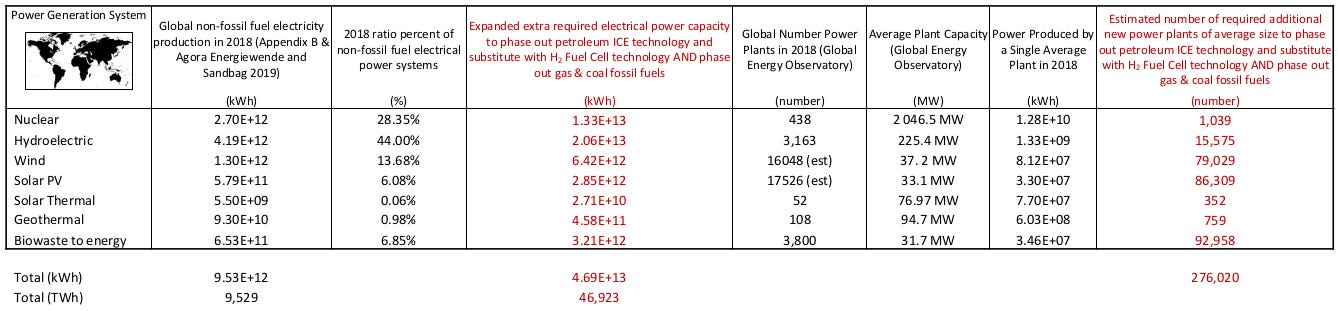
Thus, a hydrogen and renewable-only economy is more than implausible. It is impossible. At most, hydrogen fuel cells must be utilised as a long-range vehicle component for a hybrid-option future transportation fleet.
Scenario D: Biofuels or Bust!
This is the last straw, both figuratively and literally, for a clean energy transportation system. What would it take to convert the world’s fleets to utilise 100% renewable biofuels? One advantage with this transition is that combustion engines do not need replacing with electric-battery or hydrogen fuel cell motors; only the fuel changes.
Michaux dealt with a biofuel transition in chapters 21-22 (pp. 427-472). Michaux estimated that the entire planet’s biomass, 170 million tonnes, would be equivalent to approximately 94 exajoules (EJ) of energy if it was all harvested and burnt in one go. Currently, humanity utilises approximately 3.5% of that 170 million tonnes of earth biomass, mostly (62% of that 3.5%) as food production:
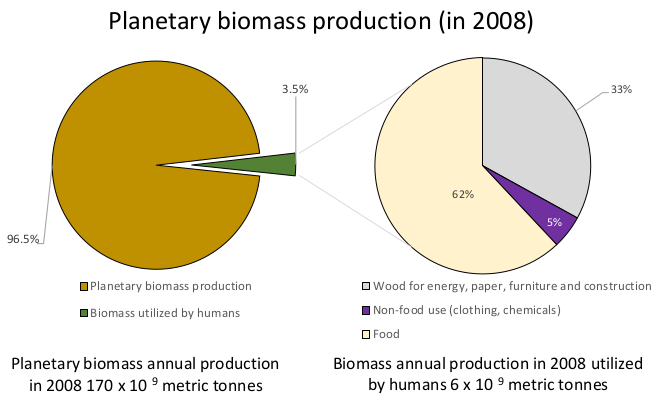
By comparison, the global energy consumption of coal, oil and gas is approximately 583.9 EJ worth of energy. At the very start it is clear that biofuels cannot ever completely replace coal, oil and gas energy production. However, Michaux considered what can be done with the limited amounts of biofuels that could be produced to replace oil alone for the transportation industry. The two most economically viable biofuels were found to be biodiesel, made from soybean, and bioethanol, made from corn (details below). The oil-based fuel benchmarked for replacement is 3.76 trillion litres:
1.48 trillion litres of petrol (US ‘gas’);
1.66 trillion litres of diesel;
263 billion litres of marine fuel oil;
359 billion litres of jet fuel.
To transition these fossil fuel sources to renewable biomass biofuels, Michaux employed the following flow chart calculation:
For biodiesel from soybeans, this was:
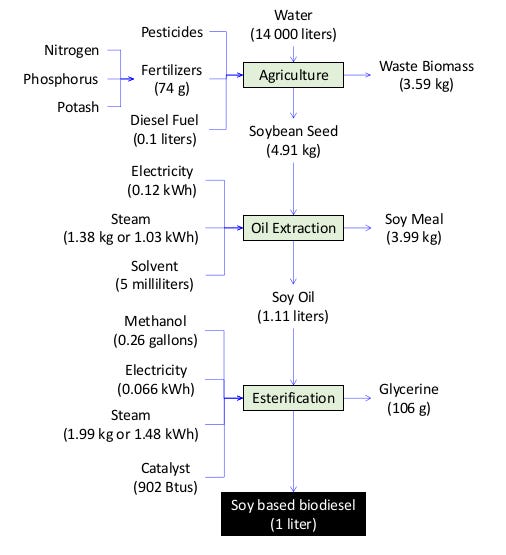
For bioethanol from corn, this was:
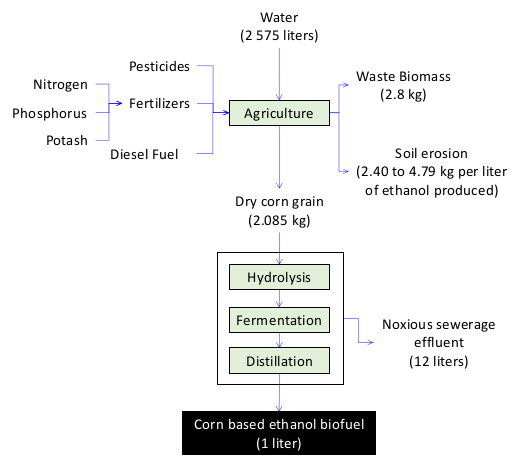
In Michaux’s estimation, 1 square kilometer of soybean would produce an average of 53,317.6 liters of biodiesel, and 1 square kilometer of corn would produce an average of 432,142 liters of bioethanol. Thus, the total land area to grow biofuels to replace all sources of fossil-fuel oil would be approximately 40.32 million square kilometers of biofuel cropland:
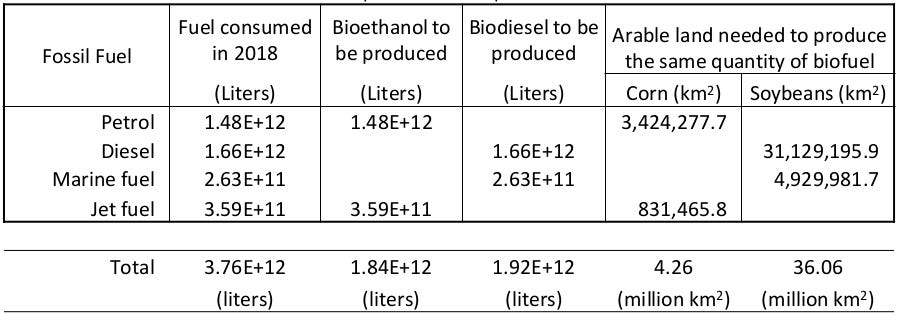
By comparison with current global land use estimates, the world’s 11 million square kilometers of arable cropland would need to be converted entirely to biofuel production. In addition to this, 74% of the world’s 39 million square kilometers of forests would have to be cleared for an additional 29 million square kilometers of arable cropland dedicated to biofuel production:
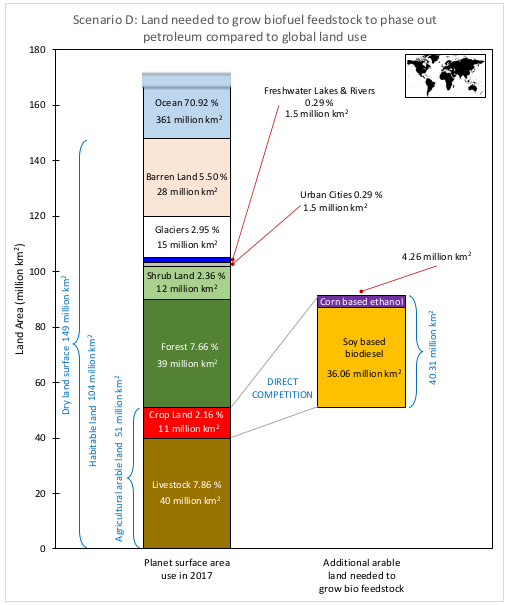
If this was not bad enough, the water consumption to irrigate those biofuel soy and corn crops would be equally obscene: 31,615.8 cubic kilometers of fresh water. Global fresh water use as of 2018 was approximately 3,972 cubic kilometers, of which existing agriculture consumes 2,760 cubic kilometers:
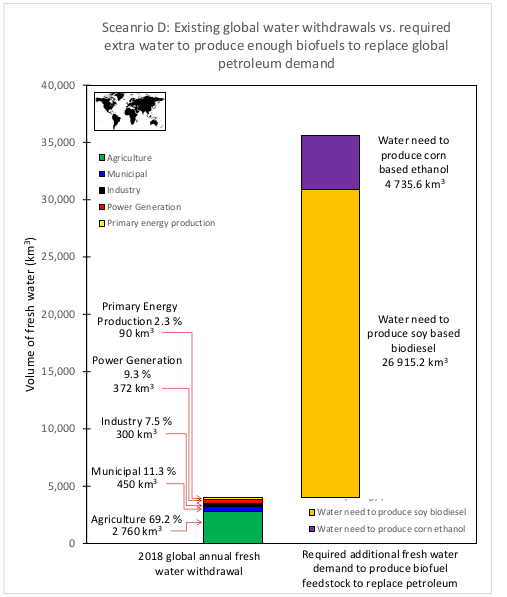
Just to be thorough, Michaux also included calculations for biofuels sourced from algae production, one of the Net Zero agenda’s hypothetical biofuel sources:
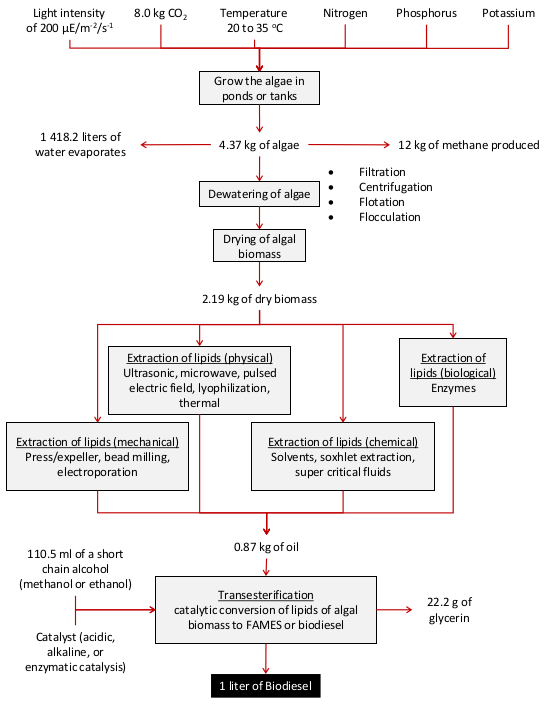
The results were predictable. To completely satisfy global oil-based fuels utilising algal-based biofuels, 1.125 million square kilometers of fresh water ponds are required. The total global open fresh water lakes and rivers is approximately 1.5 million square kilometers.
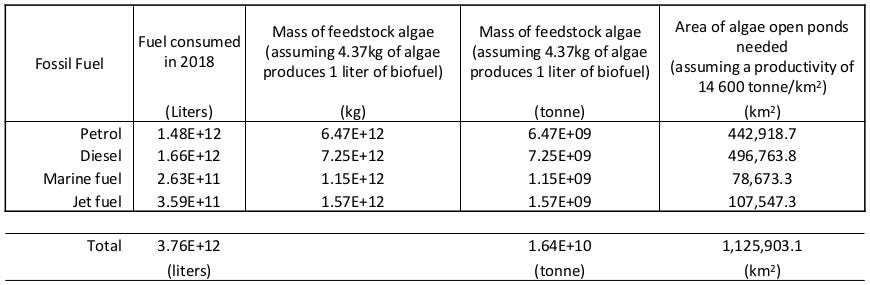
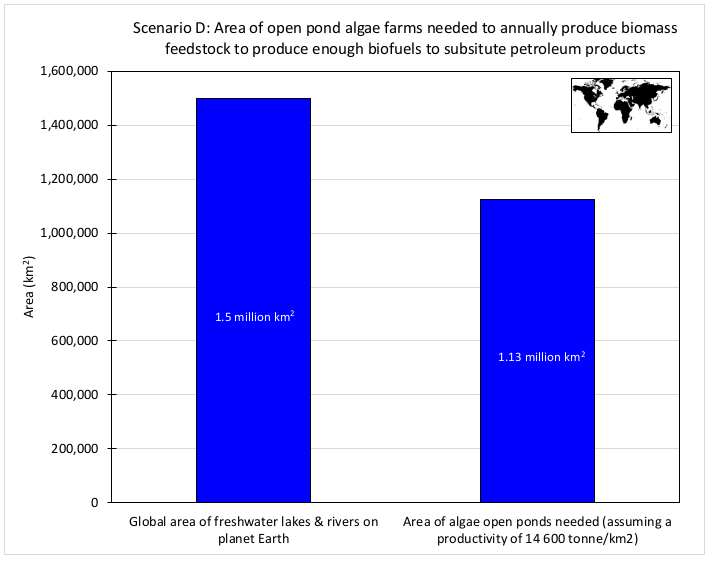
Besides the sheer impossibility of converting (or creating) 1.125 million square kilometers of fresh water algae ponds, algal biofuels entail a negative ERoEI of 0.17-0.68:1, that is, creating algal biofuels requires significantly more energy than it provides. Specifically in the global transportation context, supplying the world’s 3.76 trillion litres of oil fuels with algal biofuel would necessitate an additional 193,148.7 terawatt hours of electrical production for algal manufacturing and conversion processes, making it the worst possible option. Michaux did not even bother spelling out how many new power plants are required to supply the world with algal biofuels!
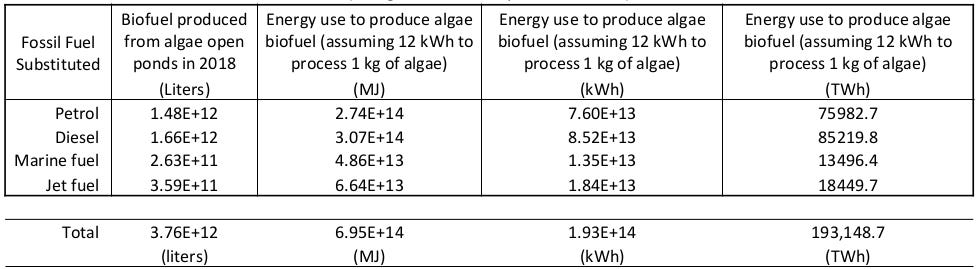
At last, we are able to make a final comparison between the three scenarios (omitted was the 40 million square kilometers of land and 31,615.8 cubic kilometers of water for soybean/corn biofuels):
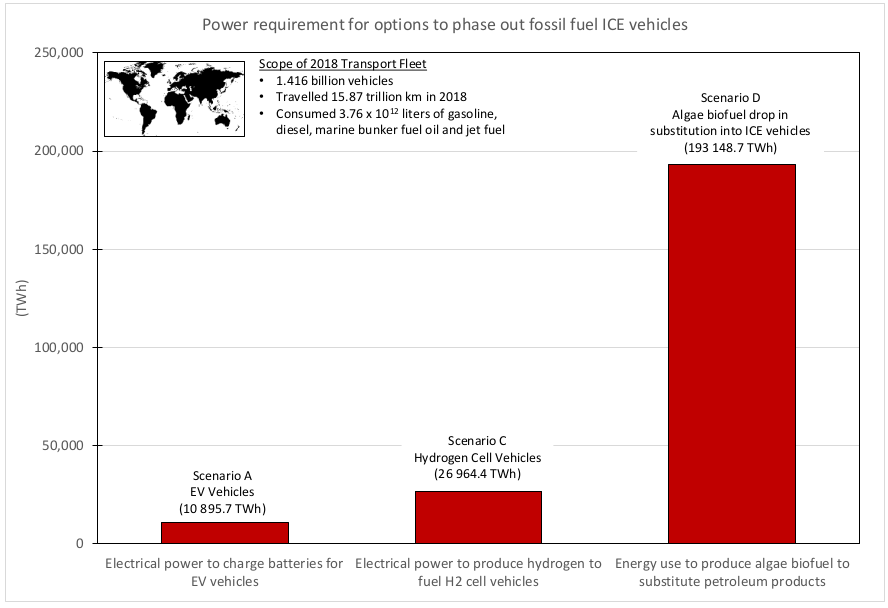
Conclusion
Michaux’s analysis clearly demonstrated that a lithium-ion battery transition is preferable for short-range transportation contexts (city passenger vehicles, city buses, short-range passenger ferries, etc). Furthermore, EVs require significantly less additional electrical power, 10,895.7 terawatt hours, than the other options. However, EV systems are highly dependent on a selection of rare earth minerals (lithium, nickel, manganese and cobalt). An important question to be answered in Part 4 is whether the world has enough of these critical minerals, 870 million tonnes, to effect an EV transition. EVs were found to be a hopeless solution for long-range freight operations such as trucks, trains and long-haul shipping; they are also entirely unsuitable for a hypothetical EV aircraft fleet due to their inherent weight. Future viability of a global EV transition will depend on the development of more efficient electric motors (greater than existing 73% efficiency), combined with more energy-dense battery chemistry (greater than 230 Wh/kg). There are several emerging options on the table which may make EV transportation fleets ultimately viable:
nuclear nanodiamond batteries that never need to be charged for their lifetime (28,000 years) and utilise otherwise unusable nuclear fuel waste as fuel;
However, and more poignantly, a 100% EV fleet would still require an additional 64,093 new non-fossil fuel power plants to power its increased energy needs, in addition to 19,900 terawatt hours of energy to phase out fossil fuel industry (e.g. steel manufacturing, plastics, chemical fertilisers), and another 17,000 terawatt hours to phase out all other fossil fuel electrical power generators. Thus, the same civil engineering nightmare we observed in Part 2 still exists for a global EV transition.
Hydrogen fuel cells were found to be a suitable replacement system for long range freight applications, especially truck, rail and shipping. The contrast in fuel weight for rail and shipping was stark: 4.32 tonnes of hydrogen vs 282 tonne lithium ion battery for a 3,000 tonne freight train; or 13,585 tonnes of hydrogen fuel vs 50,499 tonne lithium ion battery on a megafreighter travelling 8890 km. However, aircraft could not be reasonably transitioned to any current hydrogen fuel cell technology due to large pressurised hydrogen tanks occupying precious cabin space. Furthermore, the combination of phasing out coal, oil and gas sources of hydrogen and replacing them with energy-intensive electrolysis (50 kWh for 1 kg H2) to obtain the necessary 502.6 million tonnes of hydrogen to fuel for a PEM fuel cell fleet would require an additional 26,964 terawatt hours of global, non-fossil fuel energy production. This would necessitate the construction of at least an additional 158,615 new non-fossil fuel power plants to power the hydrogen economy’s vastly increased electrolysis needs. Some of these expensive energy requirements for a future hydrogen economy may be resolved by further advances in metal hydride storage systems, e.g. Magnesium hydride, and efficiency improvements in both fuel cell systems and hydrogen electrolysis. Nevertheless, the prospect of a 100% hydrogen transport economy suffers the same civil engineering nightmare: how exactly will the world construct 158,615 new renewable power plants by 2050?
Lastly, biofuels were found to be an entirely non-viable solution for 100% transition. Were the entire global transportation fleet to be transitioned away from oil-based fuels to the two most efficient biofuels, biodiesel from soybean and bioethanol from corn, it would require no less than 40.32 million square kilometers of cropland and 31,615.8 cubic kilometers of fresh water irrigation annually. When the current global cropland is 11 million square kilometers and agricultural water use is 2,760 cubic kilometers annually, this proposal becomes comical. Algal biodiesel was found to be utterly non-viable due to its inherently negative ERoEI of <1:1, as well as the impossibility of acquiring 1.125 million square kilometers of fresh water pondage. To replace the global fuel oil transportation fleet with algal biodiesel would require an insane 194,148.7 terawatt hours of new non-fossil fuel power plants. Even Michaux did not bother to calculate how many new plants this ludicrous proposal would require. That said, biofuels represent the only class of renewable energy suitable to replace jet fuel for aircraft, albeit at greatly reduced amounts. Also not mentioned above is the fact that biofuels and plant- or fungi-based plastics could potentially replace oil for the plastics industry, one of the fossil-fuel industries that cannot be phased out to anything else. Thus, biofuels may have a niche role for these two purposes in a future clean energy transition, but their resource requirements in land area and water consumption seriously detract their future viability.
What emerges is the necessity for a hybrid solution involving the best portions of each: EVs for short range transportation; hydrogen for long-range; and biofuels for aviation and plastics (covered by Michaux in chapter 26, pp. 627-655). Research and development into more efficient and energy-dense electric motors and batteries must continue. Chemical fertilisers made from oil and gas must cease and be replaced with other, fossil-fuel-free farming methods. Oil-based plastics need to be abolished and replaced with organic alternatives. But before we can even have those conversations, we need to ask a more fundamental question.
Do we have the necessary critical minerals to create an ideal, hybrid, CO2-free, clean energy transition at all? Do we have enough copper, nickel, manganese, lithium, cobalt, vanadium, uranium and other important elements to make the clean transition power infrastructure of the future? We shall discover the answer in my next installment, Part 4 - Mining the Requisite Critical Minerals for the Clean Energy Transition.
Thank you for your crystal-clear presentation of the utter impossibility of meeting Net Zero goals with any of the proposed solutions. It's abundantly clear that the only way to meet those goals is to drastically reduce the human population AND drastically reduce the standard of living of the vast majority of that population - owning nothing, and being confined to our 15 minute cities with no private motor vehicles and no commercial air travel.
The big question for me is, do our politicians know that these are the only ways to meet the Net Zero goals and they're OK with that (believing that they will be among the favoured few who will not be confined to the neofeudal plantation) or are they so stupid and ignorant that they don't understand it and are just going along with it because 'the party' says so?
Fair play for ploughing through that research!